9 Magnetic Resonance Imaging
Magnetic resonance (MR) imaging is the method of choice for identifying diseases of the bone marrow, articular cartilage, synovium, ligaments, and other soft tissues of the hand. Spin-echo (SE) and gradientecho (GRE) sequences are applied. Only the use of dedicated coils and the intravenous application of contrast medium ensure optimal image quality and high diagnostic reliability. MR arthrography is a special technique for detection of abnormal intra-articular processes. Important indications for MRI are diseases associated with trauma (fractures, ruptured ligaments, and lesions of the triangular fibrocartilage complex [TFCC]), inflammatory and tumorous diseases of the synovium, osteonecroses, and tumors of the soft tissues and bones. Apart from projection radiography, contrast-enhanced MRI is the most important technique used in hand imaging, with the exception of computed tomography (CT) for evaluating bony lesions.
MR Imaging Basics
If a patient is placed in a magnetic field, the spins of the atomic nuclei arrange themselves in this external magnetic field according to certain principles. One relates to “thermal equilibrium.” Excitation with radiofrequency (RF) energy, which is set to a particular frequency to achieve the resonance requirements, disturbs this equilibrium (the excitation process). After the source of RF energy is turned off, the system returns to equilibrium, and the body emits the supplied energy in the form of RF waves (the relaxation process). This energy is taken up by antennas (coils), and the signal is passed on for computer processing. For spatial encoding, additional spatially and temporally changeable magnetic fields (gradient fields), which are superimposed on the main magnetic field in the three spatial dimensions, are needed. Gray-scale images are calculated by special mathematical algorithms.
Changing the sequence and measurement parameters (e.g., excitation and refocusing pulses, gradient switching, repetition time [TR], and echo time [TE], inversion time [TI]), creates different image contrasts. The timing of RF pulses and gradient switching is determined by the acquisition parameters of different pulse sequences.
Only the nuclei of hydrogen-1 (1H)—i.e., protons—are used for routine diagnostic imaging of the hand in MRI. Excitation of other nuclei—e.g., sodium, potassium, fluorine—is of no importance in evaluating the hand.
Pulse Sequences
The sequence types listed in Table 9.1 are used in modern MRI systems.
One of the first sequences used in MRI was the SE sequence. This was soon followed by different GRE sequences for routine imaging, as they considerably shortened the acquisition time. Fast imaging techniques, like turbo spin-echo (TSE) or fast spin-echo (FSE), further have been developed in attempts to reduce the measurement time.
SE Sequences | GRE Sequences | ||
Conventional spinecho | SE | Conventional gradient echo | GRE |
Fast spin-echo (turbo spin-echo) | FSE, | Fast gradient echo | Various acronyms |
(Turbo)Inversion recovery | (T)IR |
Spin-Echo Technique
Depending on the repetition time (TR) and echo time (TE), spin-echo (SE) sequences can provide information about the tissue relaxation times T1 (longitudinal relaxation time) and T2 (transverse relaxation time) and thereby create different image contrasts.
A 90° excitation pulse rotates the longitudinal magnetization 90° into the transverse plane. At the same time, the spins are synchronized so that they are in phase. After turning off the excitation pulse, the transverse magnetization decreases according to the T2* decay, and the spins begin to precess at different frequencies again. This is due to the magnetic influence of their surroundings-inhomogeneity of the magnetic field caused by surrounding tissue and imperfection of the magnet.
Halfway through the echo time, a 180° refocusing pulse is applied, and inverts the spin 180° in the transverse plane, bringing the fast spins behind the slows pins. Since the spins maintain their speed and direction of rotation, the fast spins begin to catch up with the slow ones. The signal reaches its maximum at the echo time TE. This is when the signal is registered.
The 180° refocusing pulse rephases only those spins that were out of phase because of the inhomogeneity of the applied magnetic field. The spins that are out of phase because of the tissue-dependent spin-spin interaction (T2 relaxation) are not affected, so that the contrast of the different tissues is determined by the tissue-dependent relaxation times.
To determine the origin of the acquired signals, several interactions using magnetic field gradients are required:
Simultaneous application of the 90° excitation pulse and the slice-selection gradient Gs results in excitation of a given slice.
Then the phase-encoding gradient Gph is activated, which conducts one of the phase encoding steps per TR cycle.
After half the echo time, the 180° refocusing pulse is applied, and the slice-selection gradient is switched on simultaneously. The signal is read at the echo time TE. At precisely that time, the readout gradientGr is activated.
The pulse-sequence diagram for an SE sequence is shown in Fig. 9.1.
By choosing appropriate values for TR and TE, different types of contrast, so-called MRI weighting, can be achieved.
Choosing very short values for TR and TE (e.g., 600/15msec) results in a T1-weighted contrast. This contrast is determined primarily by the different T1 relaxation times. Spins in tissues with a longer T1 relaxation time cannot relax sufficiently between two excitation pulses and, therefore, do not contribute to the signal. Substances such as water appear hypointense in T1-weighted images. T2 relaxation effects are largely suppressed by the short TE.
If, in contrast, a long TR and a long TE are chosen (e.g., 3000/90msec), the result is a T2-weighted contrast. Since the spins can relax almost completely between two excitations, the signal is almost independent of T1 relaxation. Tissues with longer T2 relaxation times, like water, appear hyperintense.
If a long TR and a short TE are selected (e.g., 2200/ 17msec), contrast is largely independent of the relaxation times T1 and T2. The resulting contrast is mainly determined by the proton density.
The conventional spin-echo technique is a standard method of acquisition of T1-weighted images. Because of decreased T1 contrast and a certain amount of blurring, fast spin-echo sequences should not be used for T1-weighted imaging of the small parts of the hand.
Fast Spin-Echo Technique
Fast SE sequences are differentiated from conventional SE sequences in that several differently phase-encoded echoes are read out within a TR cycle. The echo train length (ETL) indicates the number of echoes acquired per TR cycle. The higher the echo train length, the shorter the scan time. For clinical use, echo train lengths between 3 and approximately 30 have proved useful. The FSE technique is shown in Fig. 9.2.
In spite of a contrast behavior very similar to that of the SE technique, FSE sequences display some special contrast characteristics. An obvious difference is the hyperintense representation of fatty tissue in T2-weighted images.
With the FSE technique T1-weighted, proton-density- (PD-)weighted, and T2-weighted images can be obtained. This technique can also be used for inversion-recovery sequences.
With regard to T2-weighting, the FSE sequences have replaced conventional SE sequences. In evaluation of the joints and bones of the hand, the PD-weighted FSE sequence with spectral fat saturation is useful. Not only can it identify an edematous pattern in bone marrow, but it also provides information concerning the articular cartilage. In our experience, PD FSE sequences can replace the hitherto more frequently applied STIR (short T1 inversion recovery) sequence for most clinical objectives in imaging of the hand.
Inversion Recovery Technique
Inversion recovery (IR) sequences provide excellent contrast behavior for T1- and T2-weighted images.
The IR technique additionally makes possible the acquisition of fat-saturated images, the so-called STIR sequence. It is largely identical to the SE technique, with a 180° inversion pulse added at the beginning of each TR cycle. The time from the initial 180° inversion pulse to the following 90° excitation pulse is referred to as the inversion time (TI). If the inversion time is chosen so that the longitudinal magnetization Mz of a tissue is zero, when the 90° pulse is applied this tissue cannot be excited because it is “saturated.” It is therefore displayed as hypointense in the corresponding images. In the STIR technique, the TI is chosen so that the longitudinal magnetization of the fat is zero (fat saturation). In the FLAIR (fluid-attenuated inversion recovery) technique, the longitudinal magnetization of water is zero (water saturation).
The IR technique is shown in Fig. 9.3. A disadvantage of these sequences is the long scan time, which can exceed that of SE sequences. For this reason, IR sequences are usually performed in the turbo technique.
IR sequences are rarely used in hand imaging. Only the T2-weighted STIR sequence is of importance, because its fat-suppressing ability facilitates identification of lesions containing increased fluid. However, the STIR sequence is less specific than spectral fat saturation, so the STIR technique should be used only as an alternative when spectral fat saturation provides no qualitatively satisfactory results. Since the STIR sequence can cause extinction of contrast-medium enhancement, it must not be performed after administration of a positive contrast agent.
Gradient-Echo Technique
Gradient-echo (GRE) sequences considerably shorten scan times. They have the following characteristics ( Fig. 9.4 ):
A flip angle α less than 90° can be chosen.
The flip angle α influences image contrast, as does the choice of TR and TE.
The echo is produced through inversion of the readout gradient before the signal readout; 180° refocusing pulses, like those used in the SE technique, therefore are not needed.
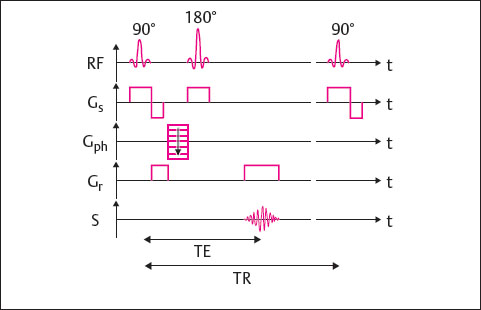
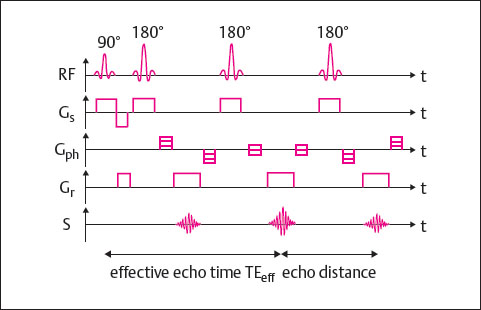
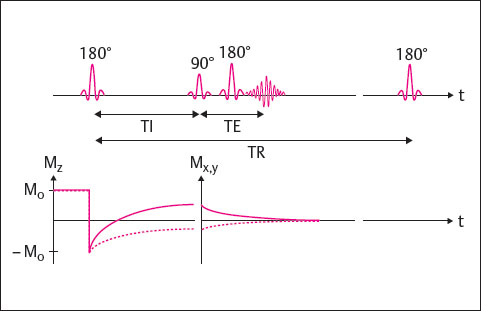
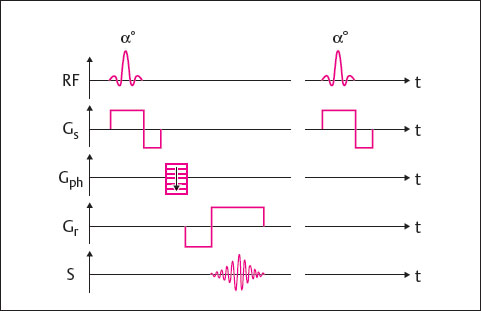
GRE sequences do not depend only on T2 effects but primarily rely on T2* decay-i.e., on T2 relaxation and the inhomogeneity of the magnetic field. For this reason, GRE sequences are highly sensitive to fluctuations in magnetic susceptibility and, therefore, to the presence of metal implants and calcifications. The specific absorption rate is significantly lower because there is no 180° refocusing pulse. Shorter times for TR and TE can be selected, thereby reducing scan times.
The following types of GRE sequences are differentiated according to their sequence designs.
GRE Sequences with Dephasing of the Transverse Magnetization
The following sequences are, in principle, similar, but have different vendor-dependent acronyms: FLASH, T1-FFE, or Spoiled GRASS.
In the FLASH sequence, at the end of a repetition cycle the phase coherence of the transverse magnetization is destroyed by a “spoiler” before the next excitation. A spoiler gradient or a suitable switching of RF pulses can serve as a spoiler and make it possible to begin quickly with the next excitation. Neither the T1 nor the T2 relaxation is complete because of the short repetition time. Using a flip angle of less than 90° reduces the signal, but with a short TR sufficient spins remain for a new excitation. Depending on the choice of the parameters TR, TE, and α, T1-weighted, PD-weighted, and T2*-weighted images can be created.
Fast T1-weighted, three-dimensional (3D) FLASH sequences are the basis of contrast-enhanced MR angiography sequences. T2*-weighted FLASH sequences in axial orientation are highly suitable for differentiation of tendons from their sheaths and, in coronal orientation, for imaging of intrinsic ligaments, as well as the components of the triangular fibrocartilage complex. No indications for T1-weighted GRE sequences of the hand have been identified.
GRE Sequences with Rephasing of the Transverse Magnetization
An example of a refocused GRE sequence is the FISP (fast imaging with steady precession) sequence (with the synonyms FFE [fast field echo] and GRASS [gradient-recalled acquisition in a steady state]). In contrast to the FLASH sequence, the transverse magnetization is not dephased but is rephased through inversion of the phase-encoding gradient.
MR angiography. FISP sequences are the basis for 3D time-of-flight (TOF) sequences. Compared with contrast-enhanced angiography sequences, 3D TOF sequences offer the advantage of higher spatial resolution. They are usually employed before administration of contrast medium.
GRE Sequences of Special Design
DESS Sequence
The DESS (dual echo steady state) technique is a double-echo, 3D GRE sequence. In the mixed sequence, two echoes with different contrasts, i.e., GRE echoes of the FISP and PSIF (reversed FISP) types, are acquired within one TR cycle. Although the PSIF part of the image is strongly T2-weighted, the FISP part of the image provides a T1/T2* contrast. The signal-to-noise ratio is very good in the DESS sequence, and multiplanar reconstruction (MPR) from the nearly isotropic 3D dataset is also possible.
Articular cartilage of medium signal intensity can easily be differentiated from joint effusion of high signal intensity with the DESS sequence. The main indication is, therefore, identification of cartilaginous lesions. If no joint effusion is present, imaging of the surface of articular cartilage is best done with MR arthrography.
MEDIC Sequence
This is a T2*-weighted GRE sequence in multiecho technique (MEDIC = multiecho data image combination). Using a FLASH sequence with flow-compensation, several echoes with different T2 weighting are collected in each TR cycle and then combined into an image.
The advantages of the MEDIC sequence are a good signal-to-noise ratio, comparatively few chemical-shift artifacts, and good T2* contrast. Initial experience has shown that the MEDIC sequence is suitable for diagnosis of cartilage abnormalities in the hand.
CISS Sequence
The CISS (constructive interference in the steady state) sequence is a 3D GRE sequence with strong T2* contrast. The CISS sequence unites two differently constructed true-FISP sequence parts.
The CISS sequence is used primarily for diagnosis of central nervous system abnormalities. In an experimental study, this sequence proved to be very well suited for imaging of the bony structures of the hand. At present, however, use of the CISS sequence is not a standard technique for diagnostic imaging of musculoskeletal structures.
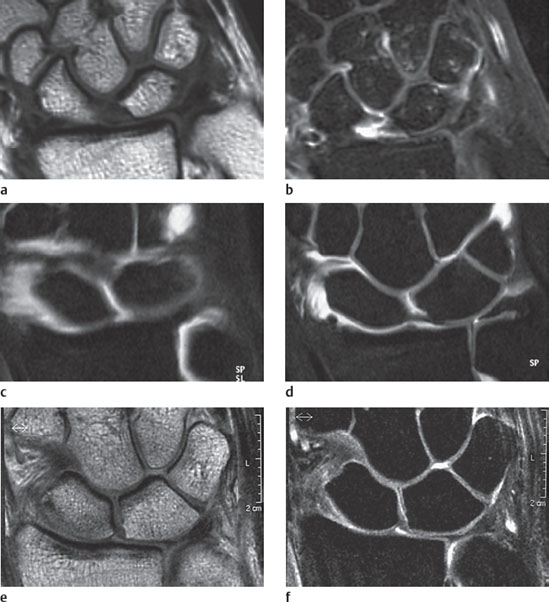
VIBE Sequence
The VIBE (volumetric interpolated breath-hold examination) sequence is a dedicated 3D gradient-recalledecho sequence. VIBE allows the volume acquisition with thin partition slices in a reasonable time due to a Fourier interpolation algorithm for symmetrically not measured phase-encoding steps in the k-space periphery. By reducing the flip angle from 12° to 15°, the VIBE sequence delivers an excellent T1 contrast. Therefore, the technique can be applied in contrast-enhanced imaging with spectral fat-saturation, as initially shown in dynamic MRI studies of the abdomen and pelvis. However, in our experience, the VIBE sequence is very useful also in 3D imaging of the wrist, particularly for depicting small intra-articular lesions with contrast-enhanced T1-weighted images of 0.5 mm thickness.
Fast and Ultrafast GRE Sequences
Fast GRE sequences, known by the names turbo-FLASH, turbo-field echo (TFE), or fast SPGR, can reduce scan times to seconds. These sequences are mainly used for dynamic contrast-enhanced studies in which the image quality is less important than short scan times.
Ultrafast gradient-echo sequences are echo-planarimaging sequences (EPI), which enable scanning to be performed within fractions of seconds. They are used primarily in neuro-MRI with diffusion, perfusion, and functional techniques.
Three-dimensional Technique (3D Technique)
In the 3D technique, an entire volume (“slab” or “chunk”) is excited, instead of a block of slices as in the 2D technique.
An advantage of volume excitation is the excellent signal-to-noise ratio. With a 3D dataset slices with minimal partition thicknesses of 0.4 mm can be acquired. For routine examinations, slice thicknesses of 1 mm are generally sufficient. Since the entire volume of the examined object is initially excited, the individual partitions must be encoded in slice-selection direction by an additional phase-encoding gradient. No gaps between the slices occur with volume excitation.
3D Sequence | Structures Displayed |
T1-weighted 3D GRE
| Articular cartilage |
T2*- and T1/T2*-weighted 3D DESS
| Articular cartilage |
T2*-weighted 3D MEDIC | Articular cartilage and bone marrow |
T2*-weighted 3D GRE | Carpal ligaments and TFCC |
T1-weighted 3D GRE
| Arterial and venous blood vessels |
A disadvantage of the 3D techniques is the relatively long scan time, and for that reason volume acquisition is useful only with GRE sequences. After data acquisition, postprocessing may be necessary and can be time-consuming. The following methods are available for postprocessing of 3D datasets:
Slices in any desired orientation, not only orthogonally aligned but also oblique or curved reconstruction slices, can be calculated from a 3D dataset by means of multiplanar reconstruction (MPR).
In MR angiographic techniques, the vessel lumen generally contains the pixels with the highest signal intensity. In the case of maximum intensity projection (MIP) only the pixels with the highest signal intensity are taken into consideration for calculation of pseudo-three- dimensional projections.
If a fixed threshold for the signal intensity is defined in image segmentation, it is possible to display the surface of the object of interest with a three-dimensional appearance from the 3D dataset (surface-shaded display [SSD]).
In volume rendering (VR) different signal intensities are correlated to defined opacity values. This procedure thus uses the information from the dataset more effectively than in the previously-mentioned techniques. Volume rendering provides high-quality three-dimensional images.
Three-dimensional techniques are used in diagnosis of the articular cartilage and the ligaments of the hand with the sequences shown in Table 9.2. Ultrafast GRE sequences are not routinely used for diagnostic imaging of the hand.
Fat-Saturation Techniques
Four different forms of fat saturation are available:
Spectral fat saturation: By selective excitation before the actual imaging procedures, the fatty tissue is selectively saturated and thereafter has reduced signal intensity. Since this technique requires a very homogenous magnetic field, it is very sensitive to artifacts and leads to inhomogeneous fat saturation if this condition is not fulfilled.
Short T1 inversion recovery (STIR): This is, in contrast to the spectral technique, a robust fat-saturation procedure that nearly always provides homogeneous fat saturation. However, it is less specific than spectral fat saturation. It can also lead to saturation of other substances when their T1 relaxation curves pass zero on the ordinate at the same time as the fatty tissue. This also applies to contrast-enhancing structures, which is why STIR sequences must not be used after administration of gadolinium. The STIR sequence cannot provide a diagnostically useful display of cartilage.
For diagnosis of the hand, spectral fat saturation is preferable to the STIR technique. The STIR technique should be used only as an alternative when spectral fat saturation is not successful.
The GRE sequence in phase and out of phase: The Larmor proton spin-precession frequencies of fat and water differ only minimally. This effect leads to a “chemical shift.” Fat and water spins are brought into phase in SE sequences by the 180° pulse at the echo time. In GRE sequences, the phases of fat and water spins depend on the echo time, since the 180° pulse is missing in this type of sequence. In this case, there are echo times in which the spins of fat and water molecules are in or out of phase. The signal behavior changes accordingly.
This sequence type is used in MRI of the abdomen. In the musculoskeletal system, the opposed-phase technique has hitherto been used only in the evaluation of changes in the bone marrow, whereby T2*-weighted opposed-phase GRE sequences can distinguish red bone marrow from abnormal bone marrow infiltrations.
Water excitation: In this technique the fatty tissue is not selectively saturated by RF pulses, but the water spins are selectively excited. As a result the water excitation acts as an indirect form of fat saturation. The value of this method remains to be evaluated in musculoskeletal MRI.
The applications and diagnostic value of the fat-saturation techniques described above are shown in Table 9.3.
Parallel Imaging
Parallel imaging is a technique to shorten scan times that is applied together with array coils. The basic idea underlying parallel imaging is that different coil elements or receiving channels of an array coil record different parts of the k-space. The spatially different sensitivity profiles of the individual coil elements are exploited. To obtain artifact-free images, the missing k-space lines must be appropriately completed. This completion can take place in the k-space, as well as in the spatial domain. SMASH (simultaneous acquisition of special harmonics) and GRAPPA (generalized autocalibrating partially parallel acquisition), for example, are methods that function in k-space, while SENSE (sensitivity encoding) works in the spatial domain. The advantage of parallel imaging is the shortening of scan time without loss of spatial resolution. This procedure does, however, reduce the signal-to-noise ratio. Besides shortening scan time, reduction of artifacts in EPI sequences is another important application of parallel imaging techniques.
Contrast Medium
Contrast Medium Effects
In contrast-enhanced MRI, positive and negative contrast agents are distinguished.
Positive contrast agents: As paramagnetic substances, these lead to signal increase of enhancing tissue in T1-weighted sequences. Since the magnetic moment of an electron shell with unpaired electrons is stronger than that of an atomic nucleus with unpaired nucleons, only substances with unpaired electrons are used as contrast agents. Gadolinium (Gd), a metal from the lanthanide group that has strong paramagnetic characteristics due to its seven unpaired electrons in half-filled 4f orbit, is most often used. In unbound form it is toxic, but this toxicity is canceled by chelate formation with DTPA or other ligands. Good contrast can be achieved with a dose of 0.1 mmol/kg body weight of gadolinium. The most important gadolinium-based contrast agents are listed in Table 9.4.
The second positive contrast agent, manganese (Mn) in the form of manganese-DPDP chelate, is of no importance in diagnosis of the musculoskeletal system.
The working principle behind paramagnetic contrast agents is a change in the relaxation times of protons that are located near the molecules of contrast agent. This leads to a reduction in relaxation times T1 and T2. Because the reduction in T1 is greater than that in T2, T1-weighted sequences are generally acquired after administration of contrast medium.
Intravenous application of contrast medium improves the contrast differences of all enhancing lesions in comparison to surrounding tissue. Two further effects can be observed in the musculoskeletal system. An intense synovial enhancement in the joints, tendon sheaths, and bursae is characteristic in the presence of synovitis. At the site of a traumatic lesion, focal enhancement occurs within the fibrovascular regenerative tissue. This enhancement can be seen about one day after the trauma in enhanced MRI and can provide decisive diagnostic information (Fig. 9.5a, b).
Negative contrast agents: These contrast agents, based on iron oxides, decrease signal intensity in T2-weighted images. One differentiates between superparamagnetic iron oxides (SPIO) and ultrasmall superparamagnetic iron oxides (USPIO). Iron oxides are preferred for diagnosis of the liver. Their value in diagnosis of bone marrow changes is currently under investigation.
Contrast Medium Administration for Standard Investigations
For standard two-dimensional (2D) imaging, a positive gadolinium-containing contrast medium is manually injected into a cubital vein via an indwelling cannula. The minimum dose for contrast medium with standard relaxivity is 0.1 mmol/kg body weight. Higher contrast can be achieved with 0.2 mmol/kg body weight (double dose). When contrast agents with a higher relaxivity or higher concentration (Multihance, Gadovist) are used, the dose can be halved.
Contrast-Enhanced MR Angiography
A 3D GRE sequence (FLASH) dataset is acquired during arterial passage of an intravenously administered bolus of contrast medium through the volume to be examined. The contrast agent is administered mechanically according to defined injection parameters with a dual-head power injector followed by a saline flush. A preinjection dataset is recorded using the same parameters. Following contrast-medium injection, the postcontrast dataset is subtracted from the precontrast dataset (filling image minus mask image). Angiographic images are computed from the subtracted dataset by maximum intensity projection (MIP).
In MR angiography of the arteries of the hand and fingers, which is described in detail in Chapter 5, a high-resolution examination technique is distinguished from a time-resolved technique.
High-resolution 3D MR angiography is performed with partitions of 1.0–1.5 mm slice thickness. The goal is to achieve nearly isotropic voxels for diagnosis of the metacarpal and finger arteries. Since scan time is between 25 and 30 seconds, exact timing of the contrast bolus with a test bolus or under fluoroscopic control is necessary. After image subtraction, MIP reconstructions in all orientations are possible.
In time-resolved 3D MR angiography, partition thicknesses of 8 mm are used. Five coronal partitions are scanned, and the result is a reduction in scan time of 3–5 seconds. If ten contiguous phases are acquired, the scan delay following contrast medium injection can be set to approximately 15 seconds. The time-resolved technique is recommended for the vessels of the forearm as far as and including the palmar arch. MIP reconstruction remains limited to the coronal acquisition plane.
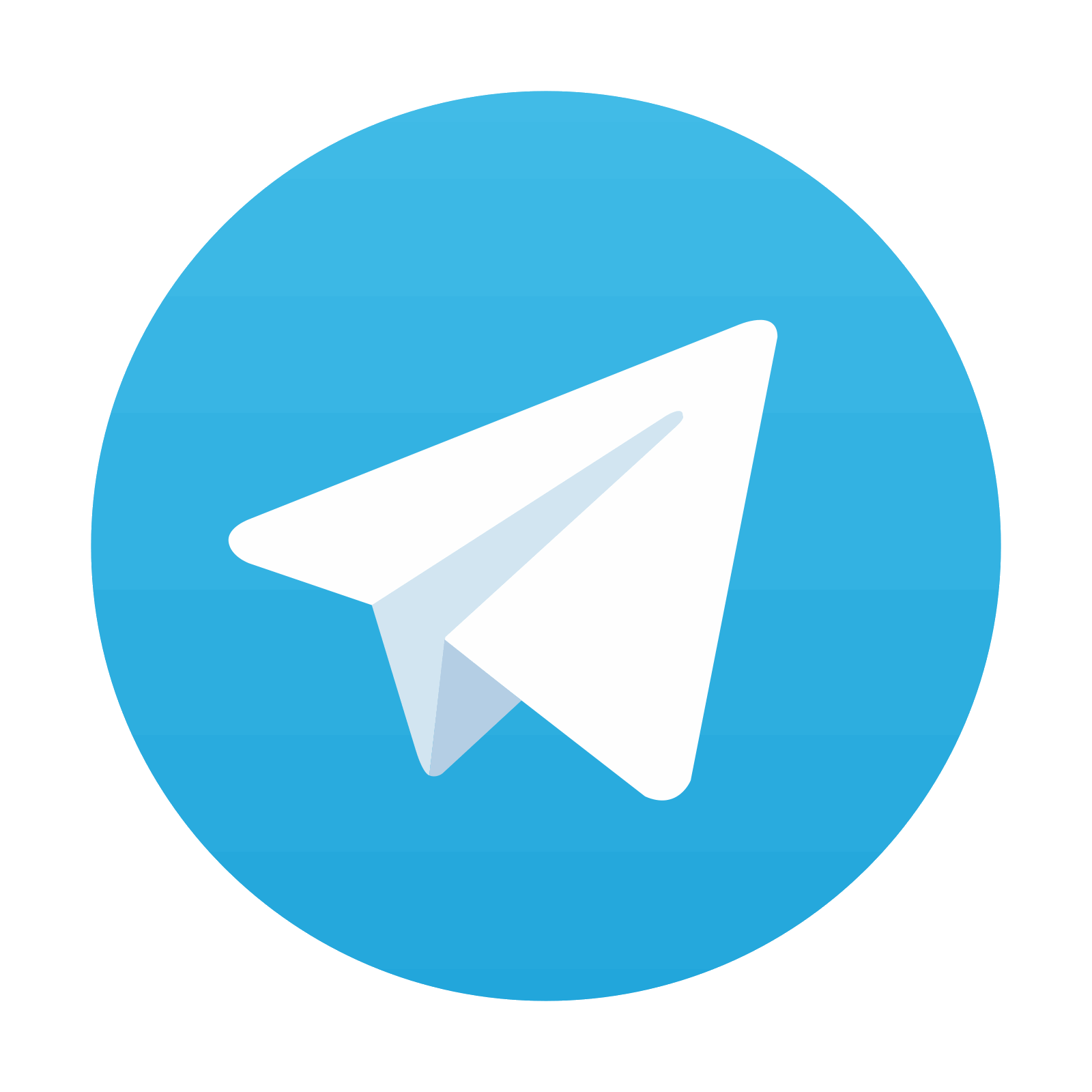
Stay updated, free articles. Join our Telegram channel
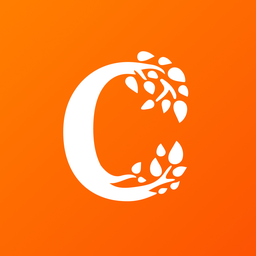
Full access? Get Clinical Tree
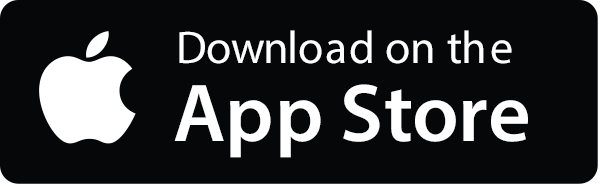
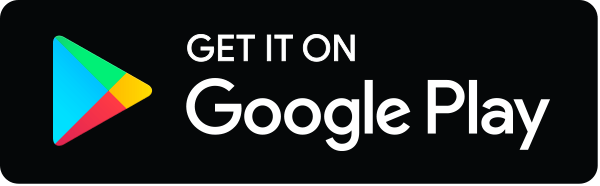
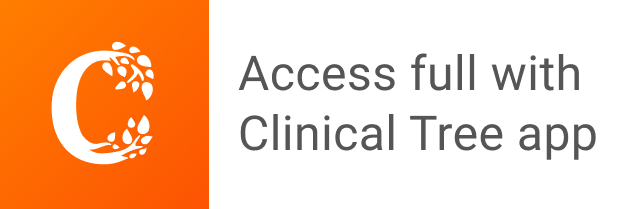