Advanced Imaging in Mild Traumatic Brain Injury and Concussion
12.1 Introduction
Mild traumatic brain injury (mTBI), sometimes referred to as concussion, can be a controversial diagnosis because imaging correlates of clinical findings and symptoms are relatively sparse on conventional computed tomography (CT) and magnetic resonance imaging (MRI) studies.1–3 These conventional imaging techniques rely on gross morphologic disturbances to manifest as visually detectable “abnormalities,” which may be identified in patients with moderate to severe traumatic brain injury (TBI) but are relatively rare in mTBI (▶ Fig. 12.1). Yet each year at least 1.7 million persons sustain TBI in the United Sates,4 leaving 80,000 to 90,000 of the survivors with significant long-term cognitive and motor disabilities.5 Many TBI survivors show significant clinical improvement over the ensuing months to years after their injury, and reliable prognostic metrics to accurately measure long-term disability are of great importance.
Fig. 12.1 Differential sensitivities across imaging techniques in detecting findings related to mild traumatic brain injury. This 12-year-old boy had sustained a concussion in a skateboarding accident. Eyewitness accounts estimate loss of consciousness (LOC) to be approximately 7 minutes, but in the emergency department the patient was alert and not amnesic. Because of the positive LOC, however, a computed tomographic scan was performed (a), followed by the more routine gradient recalled echo sequence (b), which revealed only a hint of hemosiderin deposition; however, the susceptibility-weighted sequence (c) clearly demonstrated multiple foci of hemosiderin deposition (arrows).
(Reproduced by permission from Jill Hunter, M.D., Texas Children’s Hospital, Houston, Texas. Bigler ED. Neuropsychology and clinical neuroscience of persistent post-concussive syndrome. J Int Neuropsychol Soc 2008;14:1-22.)
It is estimated that 75 to 85% of TBI is best categorized as mild, although the number of individuals who sustain mTBI is thought to be underestimated because up to 14% of mTBI patients are seen in private clinics and an additional 25% receive no medical attention.6,7 About 15 to 30% of persons diagnosed with mTBI (currently based on cognitive and clinical symptoms alone), representing the “miserable minority,”8 may be challenged by cognitive and clinical symptoms that persist beyond the first 3 months manifesting as long-term disability (i.e., postconcussive syndrome, a controversial entity).9,10 These chronic symptoms have sometimes been attributed to psychogenic causes, given the paucity of conventional radiologic imaging correlates and the relative high frequency of associated comorbidities such as depression and post-traumatic stress disorder (PTSD), which act to confound the exact underlying cause.11–13 Adding to the difficulty in quantifying the true nature and extent of the initial injury, it has been reported that conventional neuroimaging often does not reveal the brain pathology associated with mTBI.14–16 For example, in a review of four neuroimaging studies, Bazarian et al reported that 30% of TBI patients with normal CT examinations had intracranial abnormalities detected on MRI examinations.1 Other studies have suggested that MRI is more sensitive than CT in detecting lesions associated with closed head trauma and TBI.17,18 However, conventional imaging techniques are not sensitive in detecting diffuse axonal injury (DAI), which is the most commonly associated brain injury following mTBI.19 Finally, conventional CT and MRI examinations do not reliably correlate with patient symptoms and are thereby inconsistent at predicting functional outcome in mTBI.2,20
Advanced imaging methods may potentially aid in these capacities by assessing for more subtle disturbances in mTBI, both with respect to morphology, including DAI (sometimes described as traumatic axonal injury, or TAI), and with respect to physiology. Although they are still investigational, many advanced MRI techniques hold promise in their ability to better identify and quantify abnormal findings associated with mTBI. In this chapter, we focus on better defining the symptom complex attributed to mTBI and look at independent advanced MR, positron emission tomography (PET), and single-photon emission computed tomography (SPECT) imaging metrics of mTBI.
12.2 Symptoms
The two categories of mTBI are (1) complicated mild TBI, with associated positive standard neuroimaging findings; and (2) uncomplicated mild TBI, without neuroimaging findings on conventional imaging. Although the strict definition of concussion or mTBI may vary, most sources agree that “mTBI is defined as the consequence of blunt (nonpenetrating) impact with sudden acceleration, deceleration or rotation of the head with a Glasgow Coma Score (GCS) of 13–15”.21 Additional criteria often include loss of consciousness (LOC) lasting less than 30 minutes and post-traumatic amnesia lasting less than 24 hours.22 Although this list is not exhaustive, common regions of injury have historically included the upper brainstem, inferior frontal and medial temporal lobes, hypothalamic-pituitary axis, fornix, and corpus callosum (CC) (▶ Fig. 12.2 and ▶ Fig. 12.3, respectively).23 It is worth noting that autopsy reports in persons with a known history of prior mTBI (who died of unrelated causes) suggest that the CC is the most frequent site of TAI.24 Other autopsy reported areas of common involvement include the brainstem and lobar white matter.25
Fig. 12.2 Reported common areas of greatest strain effects. All views are postmortem, adapted from Mai et al (a–c) Axial views where the highlighted area represents some of the common regions where the greatest strain effects were demonstrated in studies by Bayly et al143 and Viano et al,144 respectively. (a) 1—hippocampus, 2—subiculum, 3—cerebral peduncle, 4—III ventricle, 5—hypothalamus, 6—anterior cerebral artery; (b) 7—amygdala, 8—hippocampus, 9—basilar artery, 10—temporal horn of the lateral ventricle, 11—internal carotid arteries; (c) 12—free-edge of the tentorium, 13—entorhinal cortex, 14—basilar artery, P 5 pituitary in the position of the sella turcica. (d and e) Sagittal views: 15—cerebral peduncle, 16—amygdala, 17 temporal pole; (f) A coronal view: 18—hippocampus, 19—fornix, 20—corpus callosum, 21—cerebral peduncle, and 22—entorhinal cortex adjacent to the free-edge of the tentorium. Note the closeness of all of these regions and any movement, lifting or twisting of the brain at its base would simultaneously affect all of these structures.
(Used with permission from Mai JK, Assheuer J, Paxinos G. Atlas of the Human Brain. Amsterdam: Elsevier; 2004.)
Fig. 12.3 Fiber tractography of commonly damaged tracts in mild traumatic brain injury: (a) anterior corona radiata and genu of corpus callosum, (b) uncinate fasciculus, (c) cingulum bundle in green and body of corpus callosum in red, and (d) inferior longitudinal fasciculus.
(Used with permission from Niogi and Mukherjee Niogi SN, Mukherjee P. Diffusion tensor imaging of mild traumatic brain injury. J Head Trauma Rehabil. 2010;25(4):241-55.)
Most persons suffering from concussion (i.e., mTBI) develop symptoms that may resolve within minutes, hours, or days after the injury. The term postconcussive syndrome (PCS) is usually reserved for symptoms that last more than a week after the injury.23,26 Headache and forgetfulness are the most commonly associated symptoms in the early phase of injury, often resolving by 3 months after the injury.27 However, symptoms associated with mTBI that persist longer than 3 months constitute what is commonly known as persistent postconcussive symptoms (PPCS)28–32 and can include headache, nausea, dizziness, irritability, fatigue, depression, anxiety, sleep disturbances, blurred versus double vision, hypersensitivity to light and noise, and deficits in attention, memory, concentration, executive function, and speed of processing.23 Changes in emotional regulation have also been reported.27 It is estimated that 24 to 60% of mTBI patients report one or more such symptoms at 3 months after injury or later.27,33 The reported dominant symptoms in PPCS include impaired memory, attention, and executive function, and associated changes in emotional regulation.27
12.3 Imaging Techniques
12.3.1 Diffusion Tensor Imaging
Diffusion tensor imaging, or DTI, measures the bulk directional Brownian (or random) motion of water, referred to as anisotropy. DTI has shown promise as a potential biomarker of injury in the context of mTBI, specifically related to imaging TAI, based on its sensitivity to the spatial orientation of the diffusion properties of water, notably along the direction of individual axons. When axons are injured, such as in acceleration-deceleration injuries (common in motor-vehicle collisions), the normal directionality, or anisotropy, of Brownian motion of water within axons decreases. This is due partly to restriction in axoplasmic flow versus increased flow across the axonal membrane and has been validated with animal models.34 Clinically, DTI has been shown to be more sensitive in detecting white matter injury than conventional MRI and CT, and it consistently detects more lesions than the latter two techniques, across multiple studies.35–38 Furthermore, DTI lesions have been shown to correlate with cognitive performance in mTBI.38
The platform for all DTI work is based in diffusion-weighted imaging (DWI), which is a unique imaging technique that provides physiologic information about the imaged tissue.39 DWI is a reliable and reproducible pulse sequence used to extract mean diffusivity of water on a voxel-by-voxel basis, is widely available, and can be rapidly acquired. It has been successfully implemented to routinely image the brain40,41 and is used to infer information regarding the microscopic behavior of water molecules in both the intracellular and extracellular compartments. The diffusive motion is based on the random, omnidirectional movement of free water molecules, both within and between tissues, resulting from the coupled thermal energy of these molecules, according to Fick’s law, known as Brownian motion.
In biological tissues, the otherwise omnidirectional diffusion of water molecules is hindered by tissue planes, cellular membranes, and organelles.42,43 Other factors that may play a role include cellular membrane permeability, relative size of free and bound water pools, interaction and size of the macromolecules, as well as tissue viscosity and temperature. MRI can be adapted to measure this biophysical phenomenon by application of pulsed diffusion-encoding gradients, routinely used in conjunction with a spin-echo echo-planar imaging (EPI) sequence. This method can be easily applied clinically, secondary to the rapid required imaging time, the facility of postimaging computation and generation of the diffusion maps, and the physical properties of water in biological systems. Finally, DWI is the basis for all DTI work and is of paramount importance in generating more robust and reliable DTI data. With the ever increasing number of diffusion-encoding directions as requested by some of the more advanced variants of DTI, the rapid acquisition of high-quality DWIs has become increasingly important.
Several advances have allowed for increased sensitivity in detecting the microstructural abnormalities associated with TAI. These include recent advances in DTI sequence design and diffusion tensor modeling but also include advances in MRI systems (including higher field strength), further optimization of MR coil design, and advances in image acquisition (such as parallel imaging and compressed sensing). These advances allow for increased assessment of changes related to the initial injury in mTBI, as well as reorganization and reversal of white matter changes following injury and the ability to monitor and characterize changes in brain injury over time.
As mentioned, water diffuses more readily in the orientation of the axon fibers, especially in white matter tracts, and is limited in other directions by the surrounding structural components of the axon. The degree of this directionality can be expressed as anisotropy, which is altered in the setting of TAI, thought to be related to disruption of the myelin sheath.44 The DTI pulse sequence is used to elucidate the orientation and integrity of white matter tracts by mathematically modeling the diffusion profile on a per voxel basis. A minimum of six noncollinear measurements (or directions) is required to generate a tensor model for a given voxel, which is then used to generate several descriptive scalar metrics. The most common of these include apparent diffusion coefficient (ADC); the mean diffusivity (MD) averaged over all interrogated directions; and fractional anisotropy (FA), a normalized measure that describes the degree of directionality of diffusion, assuming a Gaussian distribution. Axial diffusivity (AD), a measure of the magnitude of diffusion in the direction of the fiver orientation, and radial diffusivity (RD), a measure of the average sum of diffusivity orthogonal to the fiber orientation, are additional commonly obtained metrics. By using these parameters, healthy brain tissue is normally associated with lower ADC values and higher FA values (the latter potentially approaching unity, indicative of increased directionality and nonuniformity). In the setting of traumatic injury, expected changes include a rise in ADC values and a decrease in FA (the latter might approach zero, indicative of uniform diffusion in all directions, and loss of directionality). A summary of expected changes in DTI metrics at acute and chronic time points is presented in ▶ Table 12.1.
Acute mTBI | Chronic mTBI | |
Fractional anisotropy | ↑ | ↓ |
Radial diffusivity | ↓ | ↔ or ↑ |
Axial diffusivity | ↔ or ↓ | ↔ or ↑ |
A general review of the literature unveils four common forms of DTI analysis that are routinely used to evaluate patients with suspected acute versus chronic mTBI. These include (1) whole-brain histogram, (2) region of interest (ROI), (3) voxel-based morphometry (VBM); and (4) quantitative tractography analyses.15 Some have performed an atlas-based approach, illustrated in ▶ Fig. 12.4.
Fig. 12.4 Atlas-based regional approach: atlas with regions denoted by color to show major white matter tracts as regions of interest.
(Used with permission from Kou Z, Wu Z, Tong KA, et al. The role of advanced MR imaging findings as biomarkers of traumatic brain injury. J Head Trauma Rehabil. 2010;25:267-282.)
Of note, somewhat conflicting reports have been published describing use of the whole-brain histogram analysis, which cannot provide regionally specific information, and represents a limitation in the application of this analytical approach for prognostic utility. ROI analysis is the most commonly reported method of data analysis and is relatively easy to perform, but it is time-consuming, limited in application for individual cases, and has been criticized for possible introduction of sampling error. VBM, on the other hand, is an automated data-driven analytical method that is reproducible and able to interrogate the entire brain. The shortcomings of this technique include variable spatial smoothing and requisite (and potentially error-prone) spatial normalization and statistical correction. This technique is well-suited for group analysis, but intersubject differences are often lost in the analysis, thus reducing the apparent sensitivity for detecting differences in findings related to mTBI. Quantitative tractography is able to identify fiber tract discontinuity, which has been identified in the setting of TBI,45,46 and may hold promise as a technique to better assess loss of fiber tract coherence but remains underinvestigated in mTBI.
Older DTI pulse sequences were more prone to geometric distortion related to the slow phase-encoding bandwidth of traditional EPI sequences. Recent advances in imaging with DTI, however, has allowed for improved characterization of brain injuries in mTBI, including the ability to detect subtle changes in white matter fiber tracts and microstructural axonal injury.47,48 The use of parallel imaging, increased matrix size, motion correction, and alternate readout schemes have allowed for reduction in geometric distortion and increased fidelity of the DTI data set. However, performing DTI near the skull base, for example, is still challenged by relatively low signal-to-noise ratio (SNR) and residual geometric distortion, which reduces the reliability of tractography results because eigenvector orientation fluctuations are exacerbated considerably by low SNR. Therefore, it is imperative to monitor SNR levels and evaluate the raw DWIs or FA and color-FA maps for the presence of corruption by noise. A healthy skepticism should be maintained regarding tractography results. Furthermore, the lack of normative data and inconsistent methods of DTI acquisition (including number of directions, b-value, and voxel size) and analysis used in many studies prevent direct comparison of the respective results.49
Standard DTI analysis methods are compromised by the inability to accurately distinguish crossing fibers50 and the observation that derived values for FA erroneously assume that water diffusion has a Gaussian profile. These observations have led to the use of more complex tensor models, as suggested by Shenton et al (▶ Fig. 12.5),47 including Q-ball and other high-angular-resolution diffusion imaging (HARDI) techniques,51,52 which can provide diffusion measurements that can be used to infer the underlying tissue microstructure, such as orientation of crossing fibers. Thus, in evaluating DTI applications of mTBI, it is imperative to consider the metrics being investigated. FA, for example, is a measure derived from the diffusion tensor model, whereas f1 and f2 are derived from the partial volume model and have significant implications with respect to observed results (▶ Fig. 12.6).53,54
Fig. 12.5 Part of the corpus callosum fibers, where seeding was done in the midsagittal plane of the corpus callosum. Top figure shows the tracing using the standard single-tensor model, and bottom figure shows tracts generated with the two-tensor model.
(Used with permission from Shenton ME, Hamoda HM, Schneiderman JS, et al. A review of magnetic resonance imaging and diffusion tensor imaging findings in mild traumatic brain injury. Brain Imaging Behav. 2012;6:137-192.)
Fig. 12.6 The differences in results between the conventional [DTI] approach [fractional anisotropy (FA); top panel] and the crossing fiber approach (f1; bottom panel) show grossly consistent abnormalities; however, notable differences in methods are apparent. In particular, no voxels were detected in the brainstem for the primary fiber (bottom row), but large clusters were present there with conventional FA (top row). Skeleton voxels are highlighted in green and between group differences are in red/yellow.
(Used with permission from Mosey. Morey RA, Haswell CC, Selgrade ES, et al. Effects of chronic mild traumatic brain injury on white matter integrity in Iraq and Afghanistan war veterans. Human brain mapping, 2012.)
In a seminal study by Morey et al evaluating DTI metrics of chronic mTBI in military veterans who participated in the Iraq and Afghanistan wars (including veterans suffering from blast-related TBI), 30 subjects were compared with 42 primary and 28 confirmatory controls.55 These researchers used a HARDI diffusion model and obtained partial volume fractions for primary (f1) and secondary/crossing (f2) fibers modeled with second-order tensor modeling, followed by whole-brain voxel-wise analysis of crossing fibers as a metric of primary and secondary (crossing) fiber integrity. Their findings demonstrated that chronic mTBI was associated with loss of white matter integrity in primary fibers of major fiber bundles and smaller peripheral tracts, including the CC (genu, body, and splenium), forceps major and minor, superior and inferior corona radiata, internal capsule, superior longitudinal fasciculus, and others (▶ Fig. 12.7).
Fig. 12.7 Regarding f1 correlation with loss of consciousness, a significant correlation showed lower partial volume fraction (P < 0.05; corrected TFCE) in the primary fibers (f1) and duration of loss of consciousness in a widespread distribution of voxels in the analysis using the primary control group (top) and the confirmatory control group (bottom). Skeleton voxels are highlighted in green and correlated voxels are in blue.
(Used with permission from Mosey et al. Morey RA, Haswell CC, Selgrade ES, et al. Effects of chronic mild traumatic brain injury on white matter integrity in Iraq and Afghanistan war veterans. Human brain mapping, 2012.)
Notably, conventional three-dimensional (3D)-FSPGR (Fast Spoiled Gradient Echo) T1-weighted images failed to reveal any abnormalities.55 They reported that the distributed loss of white matter integrity correlated with subjects’ “feeling dazed or confused” and, to a slightly lesser extent, with duration of LOC, but not with the diagnosis of PTSD or depressive symptoms.55 In conclusion, compared with DTI, the measured variable, f1, was more specific to mTBI than DTI-derived FA.
Serial short-term DTI assessment of brain structural changes were performed by Wilde, McCauley, and colleagues in evaluating eight subjects with uncomplicated mTBI over the first 8 days after injury.56 Imaging metrics, including DTI-derived FA, ADC, AD, and RD (of the left cingulum), at four time points were compared with neuropsychological testing and memory performance (via Hopkins Verbal Learning Test—Revised). The DTI sequence acquired 70 slices, obtained with 30 diffusion-encoding directions, a sensitivity encoding reduction factor of 2, and averaged two combined acquisitions to improve the SNR. The results of this study demonstrated that memory performance was transiently affected throughout the week, was most affected at the second assessment (between days 3 and 4 or 97 to 144 hours after injury), and returned to baseline by 8 days after injury. FA transiently increased in some participants, but the pattern and degree of symmetry between FA and memory performance were complex and did not always correlate.56 Although the authors cite the small sample and short imaging interval as limitations to the study, the results highlight the degree of complex variability within and between subjects, depending on time of imaging post injury, as demonstrated in ▶ Fig. 12.8. Although several confounding variables are acknowledged, these results support the overall assumption that most patients with sports-related mTBI may exhibit transient symptoms that appear to spontaneously and completely resolve within 2 to 14 days after their injury57–60 and up to 3 months after alternate mechanisms of injury.61 A subset of subjects may demonstrate PPCS beyond 3 months, as previously described.
Fig. 12.8 Line graphs illustrating measurement of (a) fractional anisotropy (FA), (b) apparent diffusion coefficient (ADC), (c) axial diffusivity (AD), and (d) radial diffusivity (RD) in the left cingulum bundle over the four assessment occasions in each participant. Comparison of the trajectories of apparent diffusion coefficient (b), axial diffusivity (c), and radial diffusivity (d) for each participant highlights the complex and variable nature of change as measured by diffusion tensor imaging. Considerable qualitative differences in the pattern of change are noted between metrics and between subjects, in which some metrics indicate a more prominent change within each individual, varying by the metric used.
(Modified from Wilde EA, McCauley SR, Barnes A, et al. Serial measurement of memory and diffusion tensor imaging changes within the first week following uncomplicated mild traumatic brain injury. Brain Imaging Behav. 2012;6:319-328.)
In evaluating severe TBI with DTI, Betz et al revealed prognostic value in whole-brain and regional DTI measures.62 The authors evaluated DTI measures of ADC, FA, axial (AD), and radial diffusivity (RD) from whole-brain white matter, internal capsule, genu, splenium, and body of the corpus callosum compared with neurologic status at MRI and at discharge to acute TBI rehabilitation and demonstrated a significant correlation with GCS score on the day of MRI examination for whole-brain white matter averages of ADC, AD, and RD and their coefficient of variance.62
Similar results have been demonstrated in evaluating mTBI with DTI. A systematic meta-analysis of the application of DTI in mTBI was performed by Aoki et al63 in evaluating 13 independent DTI studies investigating changes in FA and MD involving regions of the CC. These researchers used a random-effect model and revealed significant reduction in FA with a concomitant increase in MD involving the CC in 280 mTBI patients and 244 controls. When evaluating subregions of the CC, their results suggested a significant reduction in FA and a significant increase in MD within the splenium of the CC, as determined by one-way sensitivity analysis of each meta-analysis. Interestingly, FA was identified as only marginally reduced in the midbody of the CC, and no significant change in FA or MD was identified within the genu of the CC. Given the hypothesis that linear and angular acceleration may damage callosal fibers, evaluating changes in FA and MD demonstrated that the posterior portion of the CC (including the splenium) was more vulnerable to mTBI compared with the anterior portion.63
Studies evaluating mTBI with DTI have demonstrated significant associations between aberrant white-matter anisotropy and various functional outcome measures.64–67 Because of this observation, differences in findings between studies have often been attributed to differences in methods. However, an additional degree of spatial variability is present even when comparing studies similar in design and methods, suggesting possible inherent heterogeneity in the distribution of pathology, exemplified by VBM studies in mTBI. This is typified by one such study that identified regions of decreased FA involving the subcortical white matter, centrum semiovale, CC, internal capsule, and deep cerebellar white matter in both group and individual analyses.36 Using a similar VBM analysis, another study evaluating chronic mTBI demonstrated relative sparing of the internal capsule,46 whereas yet a third study demonstrated involvement of the external capsule.64 Interestingly, the above-mentioned meta-analysis by Aoki et al demonstrated that decreased FA in the spenium of the CC represented the most frequently injured region of the CC,63 confirmed by ROI studies such as that performed by Inglese et al evaluating uncomplicated mTBI at both acute and chronic time points post injury.2 However, Niogi et al38 evaluated complicated and uncomplicated mTBI with ROI analysis and concluded that the genu of the CC rather than the splenium, was among the most frequently injured brain region, based on decreases in FA. Such discrepancies are illustrative of this pathological heterogeneity, which may be exacerbated by inclusion of acute and chronic, as well as uncomplicated and complicated mTBI. These observations are further complicated by the challenges inherent in the differing methods of DTI data acquisition and quantification, which include but are not limited to differences in head coil, number of gradient directions, b-value, voxel size, scanner manufacturer, software platform, and field strength.
In conclusion, the currently used methods for obtaining and processing DTI data sets suggest that FA and other commonly DTI-derived metrics are sensitive in detecting changes associated with mTBI and appear to be most sensitive in the posterior region of the CC, although some disparity between involved structures and their frequency of involvement is acknowledged. Whereas the sensitivity of DTI in detecting mTBI-related abnormalities is reassuring (▶ Fig. 12.9), the necessary longitudinal studies to evaluate the prognostic contribution of DTI in the workup and management of mTBI have yet to be performed. Moreover, the caveat that FA assumes that water diffusion has a Gaussian profile cannot be overstated; this likely represents an erroneous oversimplification of the derived tensors, which may be sensitive to acutely injured fiber tracts in the setting of mTBI but may not be specific in quantifying prognosis. Use of more complex tensor models will likely become more commonplace in the near future to improve specificity and microstructural assessment of crossing fibers and fiber tracts in the setting of mTBI. Finally, the analytical model used to evaluate both individual DTI data and group data sets provide disparate information, each with their own caveats. The most beneficial DTI metrics in evaluating mTBI have yet to be determined given these multiple challenges. Nonetheless, DTI appears to provide added value in allowing for potential imaging correlates to the clinical observations made in patients suffering from acute mTBI. Continued optimism in this technique in this capacity is certain to entice additional research in this arena.
Fig. 12.9 Diffusion tensor images captured on a 3 T magnet analyzed with streamline tractography using Slicer 3. Control brain on the left and the brain of a former professional boxer in his 40s on the right. The top two images are sagittal views with the callosal fiber tracts delineated; it is notable that the boxer’s fiber tracts are markedly shorter than the control. The bottom two images are a coronal view of the same two individuals, and it can be seen that the athlete’s corpus callosum (red structure in the middle of the brain) is noticeably thinner than the control.
(Used with permission from Baugh. Brain Imaging and Behavior (2012) 6:244–254.)
12.3.2 Single-Photon Emission Computed Tomography
SPECT is a functional neuroimaging technique used to measure cerebral blood flow based on the distribution of intravenously injected radiopharmaceutical. Tomographic images representing the cerebral activity of the radiopharmaceutical are produced after the capture of emitted photons via 360-degree rotation of a gamma camera about the subject. 3D images are then constructed from this tomographic data set. The benefits of this technique include general availability to perform the examination at most hospitals, relative low cost of radionuclides compared with those of PET, longer radionuclide half-life (generally using technetium-99m-hexamethylpropylene amine oxime [99mTc HMPAO]), and general increased clinical availability of the device.
SPECT is a sensitive tool for detecting regional abnormalities in brain function and relative cerebral blood flow (CBF), but it differs from PET in that SPECT studies typically measure the brain at rest. In this context, measured CBF is considered an indirect gauge of brain metabolism in healthy brain tissue, which may not hold true in the setting of TBI.68 Most SPECT imaging relies on comparisons between several ROIs to assess for regional differences in blood flow, which must be compared with regions that are presumably free of injury and may be difficult in the setting of TBI given the potentially diffuse nature of the injuries. Finally, the specificity of SPECT has been described as relatively poor.69 Although a recent study comparing SPECT findings in retired National Football League players with those of age-matched controls reported significant differences,70 the study methods have been called into question, making the results difficult to interpret.
Nonetheless, SPECT offers promise in evaluating mTBI given its clinical feasibility and increased sensitivity in detecting abnormalities in the setting of mTBI compared with conventional MRI (▶ Fig. 12.10).71 The longitudinal predictive power of SPECT was demonstrated in a larger study by Jacobs et al that evaluated 136 patients for 1 year who presented with a GCS greater than 13 and normal admission CT head examination.72 These researchers reported a conditional probability of a poor clinical outcome with abnormalities detected on initial SPECT scan of 44% at 3 months and 83% at 12 months, and they reported a favorable clinical outcome with initial normal SPECT scan of 92% at 3 months and 100% at 12 months. The high number of false-positive results at 3 and 6 months (based on the predicted poor outcome given presence of abnormal finding on initial SPECT scan, which were not supported at 12 months) led these researchers to caution against predicting a poor recovery when initial SPECT scan is not interpreted as normal.72 The observation that a normal initial SPECT scan is highly suggestive of a good clinical outcome has led the American Academy of Neurology to implement guidelines that recommend that SPECT imaging be used as an investigational tool only because the significance of “positive” findings is not known.73
Fig. 12.10 Images obtained from a 37-year-old bicyclist. (a) Single-photon emission computed tomography image shows a right frontal perfusion deficit. (b) Corresponding fluid-attenuated inversion recovery (FLAIR) image shows a hemorrhagic contusion.
(Used with permission from Hofman PA et al. Am J Neuroradiol 2001;22:441-449.)
Although the number of studies performed examining the utility of SPECT imaging as applied to mTBI is beyond the scope of this chapter, most SPECT studies performed within the first few weeks after injury reveal hypoperfusion associated with acute mTBI (notably in the frontal and temporal lobes).71,74,75 However, the prognostic and clinical value and the utility of abnormal findings on initial SPECT imaging were either not demonstrated,71 reported qualitatively,74 or not reported. Studies performed to evaluate chronic mTBI (6 months or more post injury) usually focused on symptomatic subjects and have also demonstrated hypoperfusion on SPECT imaging (generally involving the frontal and temporal lobes), but the relationship between findings identified on SPECT imaging and neuropsychological testing as well as reported symptoms and the clinical picture remain inconsistent.76–80 Future studies are required to better answer these questions.
12.3.3 Positron Emission Tomography
PET imaging uses positron emitting tracers (most commonly 2-deoxy-2-(18F)-fluoro-D-glucose [FDG]) that annihilate with electrons within millimeters from their site of emission. This annihilation results in two gamma photons being emitted at 180 degrees relative to one another, which are subsequently temporally detected in “coincident” fashion via rings of radiation detectors in the gantry of the PET scanner. The coincident detection provides higher spatial resolution than SPECT images (usually on the order of 1 cm). FDG PET assesses regional brain glucose metabolism since the FDG ligand behaves much like glucose in the body, which crosses the blood-brain barrier and is taken up and trapped by the brain cells. In this capacity, the trapped FDG (a nonmetabolized molecule) can be used for glucose metabolic imaging to evaluate for local brain damage, providing information that is not significantly dissimilar from that obtained with 99mTc HMPAO SPECT. However, the PET isotopes have a shorter half-life than those used in SPECT imaging and require that the radiopharmaceutical be more readily available, increasing the cost of the examination.
For these reasons, few studies have been performed with FDG PET in mTBI. In fact, no acute mTBI cohort FDG PET studies have been published to date. Of the chronic mTBI studies performed with FDG PET, all evaluated mTBI months to years after the initial injury. Early studies provided quantitative corroboration of neuropsychological testing in attention and memory such that mTBI subjects demonstrated significantly worse performance, but these studies were faulted for lack of direct correlation between PET measures with testing results.81,82 In a separate study evaluating 20 chronic mTBI patients, a total of 82 ROIs were compared, revealing foci of hypometabolism within the midtemporal lobe in three patients and foci of hypermetabolism within the midtemporal lobe in another 12 patients.83 Similar contradictory findings were identified in other brain regions, although all 20 subjects demonstrated some notable FDG PET abnormalities. Surprisingly, the combination of these presumably discordant findings correlated with neuropsychological testing.83 Combined PET-SPECT studies have revealed similar contradictory findings such that one study identified changes in the bilateral temporal lobes,80 whereas a similar study found no such changes.84
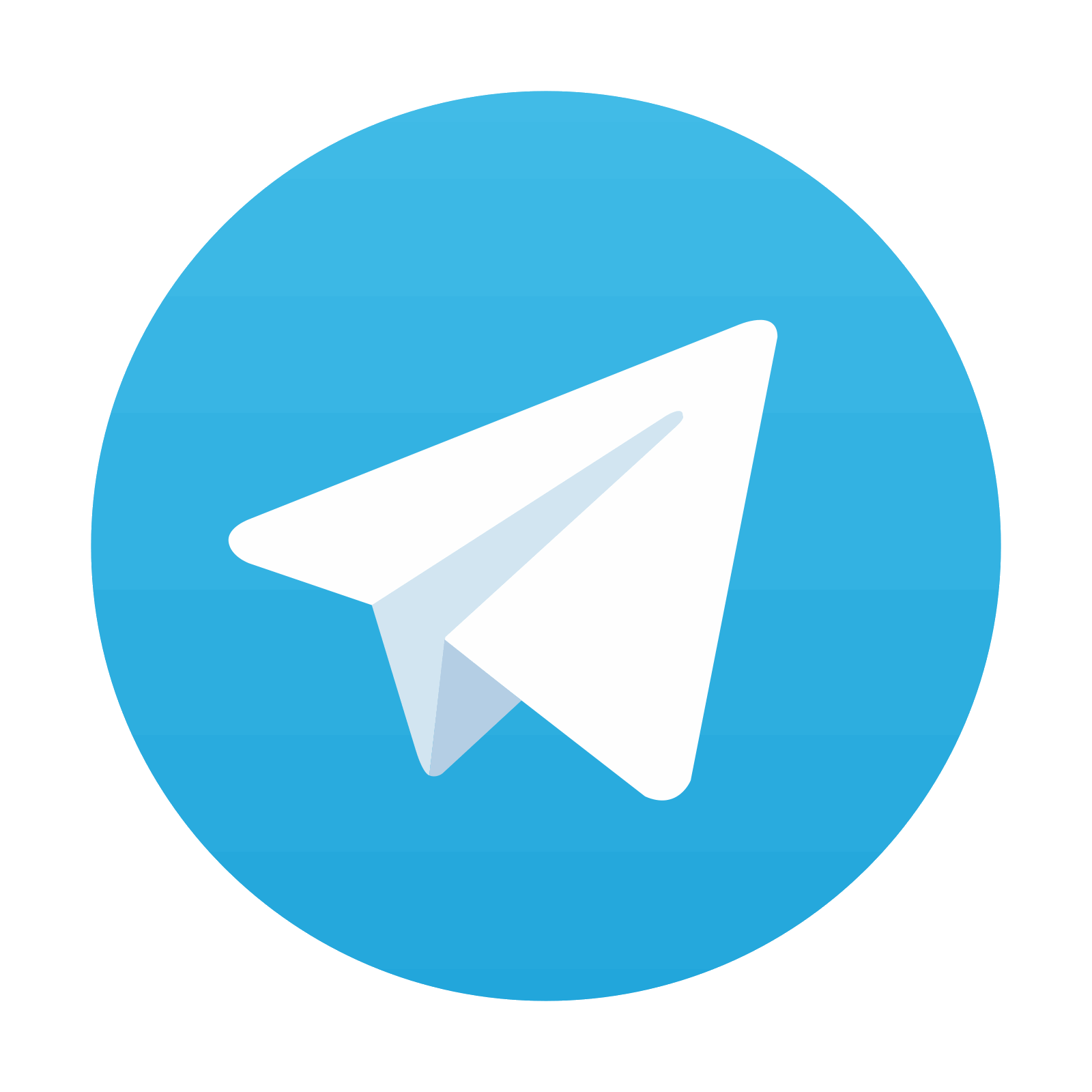
Stay updated, free articles. Join our Telegram channel
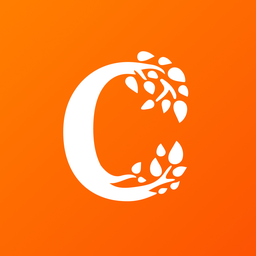
Full access? Get Clinical Tree
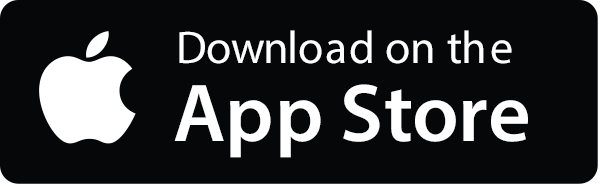
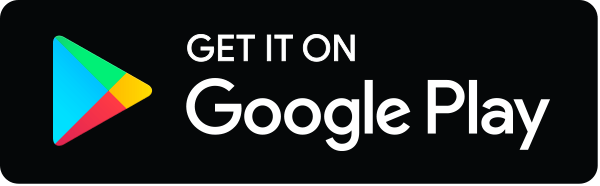