Alternative Radiation Modalities
The early recognition that x-rays could produce local tumor control in some patients and not in others led to the notion that other forms of ionizing radiations might be superior.
Neutrons were first introduced in a speculative way, not based on any particular hypothesis. The later use of neutrons and the introduction of protons, negative π-mesons, and heavy ions were all based clearly on a putative advantage, either of physical dose distribution or of radiobiologic properties. In the case of neutrons, they give up their energy to produce recoil protons, α-particles, and heavier nuclear fragments. Consequently, their biologic properties differ from those of x-rays: reduced oxygen enhancement ratio (OER), little or no repair of sublethal damage, and less variation of sensitivity through the cell cycle.
The use of neutrons following World War II was based squarely on the premise that hypoxic cells limit the curability of human tumors by x-ray therapy, so the lower OER characteristic of neutrons might confer an advantage. An alternative rationale for neutrons, proposed at a later date, was that their relative biologic effectiveness (RBE) is larger for slow-growing tumors, possibly giving them an advantage in a limited number of specific human tumors.
Protons have radiobiologic properties similar to those of x-rays, and their introduction into radiotherapy was based entirely on the superiority of the physical dose distribution possible with charged particles. Negative π-mesons and heavy ions were introduced with the hope of combining the radiobiologic advantages attributed to neutrons with the dose distribution advantage characteristic of protons.
Neutrons have been shown to be superior to x-rays in a limited number of situations, specifically for the treatment of prostatic cancer, salivary gland tumors, and possibly soft tissue sarcomas. Several controlled clinical trials have been performed for various cancer sites, but a small gain was apparent only in these few circumstances. On the other hand, neutrons resulted in unacceptable late normal tissue damage in many cases, and possibly a higher incidence of radiation-induced second cancers.
For almost 50 years, protons generated in facilities originally intended for physics research have found a small but important niche for the treatment of uveal melanoma and tumors such as chordomas, which are located close to the spinal cord and therefore benefit greatly from the localized dose distribution. The wider use of protons for broad beam radiotherapy is being tested now that custom-built hospital-based facilities are available, but no advantage has been demonstrated yet in controlled phase 3 clinical trials.
Negative π-mesons and heavy ions have been used to treat hundreds of patients, but prospective randomized trials have never been completed to prove their superiority over conventional x-rays. Negative π-mesons have completely disappeared
from the scene, but high-energy carbon ions are enjoying a renaissance in Europe and Japan.
from the scene, but high-energy carbon ions are enjoying a renaissance in Europe and Japan.
The casual reader may be content with this overview of alternative radiation modalities and may not wish to proceed further in this chapter. Interest in high-linear energy transfer (LET) radiations for radiotherapy largely has waned, but protons are very much in vogue. In this chapter, neutrons, protons, and carbon ions are considered in turn.
▪ FAST NEUTRONS
Rationale
Neutrons were first used for cancer therapy at the Lawrence Berkeley Laboratory in California in the 1930s (Fig. 25.1). This first clinical trial of neutrons was not based on any radiobiologic rationale but was prompted largely by the availability of a new and unique beam. It is said that it received some impetus when the mother of the Lawrence brothers (E.O. Lawrence was the inventor of the cyclotron and the director of what was to become the Lawrence Berkeley Laboratory) contracted cancer, which was judged by her physician to be incurable by conventional means. She was treated with neutrons and lived for many years, although from a retrospective review of the case, it is probable that she did not have cancer in the first place. This early effort at Berkeley was hampered because the complexities of the relationship between the RBE and the dose for high-LET radiations were not understood at the time. Consequently, several patients were overdosed seriously before the trial was terminated by the entry of the United States into World War II. In reviewing their experience many years later, Dr. Robert Stone, the radiotherapist in charge of the study, concluded in his famous Janeway Lecture of 1948 that “Neutron therapy as administered by us resulted in such bad late sequelae in proportion to a few good results that it should not be continued.”
The Hammersmith Neutron Experience
The renewed interest in neutrons in the years following World War II originated at the Hammersmith Hospital in London, where neutrons were generated by the Medical Research Council’s 60-in. cyclotron. In this machine, 16-MeV deuterons incident on a beryllium target produced neutrons with a modal value of 6 MeV.
The Hammersmith cyclotron was suggested and conceived by Gray, based on the notion that a lowered OER would be advantageous to radiotherapy. The machine suffered from the limitations of poor depth doses (equivalent to 250-kVp x-rays) and a fixed horizontal beam.
The Hammersmith cyclotron was suggested and conceived by Gray, based on the notion that a lowered OER would be advantageous to radiotherapy. The machine suffered from the limitations of poor depth doses (equivalent to 250-kVp x-rays) and a fixed horizontal beam.
A prospective randomized clinical trial to compare neutrons with x-rays was started in 1971. Advanced tumors of the head and neck were chosen because the poor depth-dose characteristics made neutrons suitable only for treating relatively superficial lesions. The trial involved patients with tumors of the salivary glands, buccal cavity, hypopharynx, and larynx. The neutron treatments delivered in only 12 fractions were clearly superior as judged by local control of the primary tumor, but the gain was achieved at the expense of a higher complication rate.
The United States Neutron Experience
Because of the limitations associated with low-energy cyclotrons, interest at several centers in the United States turned to the use of large cyclotrons that accelerate deuterons to energies of 22 to 50 MeV. Several such machines had been built for high-energy physics research and were converted for part-time neutron therapy. In addition, neutrons were produced at the Fermilab in Batavia, Illinois, by bombarding a beryllium target with 67-MeV protons.
All of these machines had adequate dose rates and quite good depth doses. Unfortunately, they had other disadvantages: All had fixed horizontal beams, and all were located in physics installations rather than in large, busy hospitals, so that the availability of a sufficient number of patients was a problem. Several controlled clinical trials were performed for various tumor sites, and they showed no advantage for neutrons over x-rays. Neutrons, however, appeared to be superior for salivary gland tumors, soft tissue sarcomas, and prostate cancer, but the downside was a significant increase in late normal tissue damage. It appears that high-LET radiation loses the differential sparing effect between tumors and lateresponding normal tissues that is characteristic of x-rays.
Enthusiasm for neutron therapy waned just at a time when technology became available that allowed machines to be built that are suitable for clinical use. A new generation of hospital-based cyclotrons using the p+ → Be reaction had adequate dose rates, good percentage depth doses, and a full isocentric mount, similar to a conventional linac. A few centers operated such machines for a while, but neutrons have never become mainstream in radiotherapy.
▪ BORON NEUTRON CAPTURE THERAPY
The basic idea behind boron neutron capture therapy (BNCT) is elegant in its simplicity. It has appealed to physicians, and particularly to physicists, for the best part of half a century. The idea is to deliver to the cancer patient a boroncontaining drug that is taken up only in tumor cells and then to expose the patient to a beam of low-energy (thermal) neutrons that themselves produce little radiobiologic effect but that interact with the boron to produce short-range, densely ionizing α-particles. Thus, the tumor is intensely irradiated, but the normal tissues are spared. There are two problems inherent in this idea that have so far proved intractable:
How does one find a “magic” drug that can distinguish malignant cells from normal cells? (The skeptic might add that searching for such a drug has been the holy grail of cancer research and that if one were found, the obvious strategy would be to attach an alkylating agent or an α-emitting radionuclide to it; combining its use with neutrons would be a distant third.)
The low-energy neutrons necessary for BNCT are poorly penetrating in tissue and consequently result in percentage depth doses that are extremely poor by today’s standards.
Several nuclides have high propensities for absorbing low-energy or thermal neutrons; that is, they have a high neutron capture cross section. Boron is the most attractive of these because it is readily available in a nonradioactive form, its chemistry is such that it can be incorporated into various compounds, and if it interacts with low-energy neutrons, it emits short-range, high-LET α-particles.
Boron Compounds
For BNCT to be successful, the compounds used should have high specificity for malignant cells, with concomitantly low concentrations in adjacent normal tissues and in blood. In the
early days, the compounds used were not specially synthesized for BNCT, but were already available. In the brain, which is the site for which BNCT largely has been used, some selectivity is obtained because compounds do not penetrate normal brain tissue to the same degree as brain tumors in which the blood-brain barrier is absent or severely compromised.
early days, the compounds used were not specially synthesized for BNCT, but were already available. In the brain, which is the site for which BNCT largely has been used, some selectivity is obtained because compounds do not penetrate normal brain tissue to the same degree as brain tumors in which the blood-brain barrier is absent or severely compromised.
Critical to the success of BNCT is the requirement that boron compounds be developed that target tumor versus normal cells selectively, achieve a sufficient concentration within the tumor, and produce tumor to normal tissue ratios of 3 or 4 to 1. This, of course, is a tall order.
Two classes of compounds have been proposed:
Low-molecular-weight agents that simulate chemical precursors required for tumor cell proliferation have the ability to traverse the cell membrane and be retained intracellularly. Two boron compounds have been identified and used clinically, known as sodium brocaptate and boronophenylalanine. Both have been used to treat brain tumors, and the latter also has been listed for cutaneous melanoma.
High-molecular-weight agents such as monoclonal antibodies and bispecific antibodies that contain boron have been developed. These are highly specific, but very small amounts reach brain tumors following systemic administration. Boron-containing conjugates of epidermal growth factor, the receptor for which is overexpressed on some tumors (including glioblastoma), also have been developed.
If the blood-brain barrier is disrupted temporarily, these high-molecular-weight compounds may have some utility, or direct intracerebral delivery may be required. They have not yet proved effective in clinical use.
Neutron Sources
During fission within the core of a nuclear reactor, neutrons are “born” that have a wide range of energies. Neutron beams can be extracted from the reactor by the application of suitable techniques and the use of appropriate moderators. Thermal neutrons, or room-temperature neutrons (0.025 eV), react best with boron to produce densely ionizing α-particles. Unfortunately, thermal neutrons are attenuated rapidly by tissue; the half-value layer is only about 1.5 cm. Consequently, it is not possible to treat to depths of more than a few centimeters without heavily irradiating surface normal tissues. Nevertheless, most clinical trials have used neutrons of this energy.
Current interest in the United States focuses on the use of epithermal neutron beams (1-10,000 eV), which have a somewhat greater depth of penetration. These can be obtained by using moderators or filters to slow the fast neutrons into the epithermal range and filtering out the residual thermal neutrons. These epithermal neutrons do not interact themselves with the boron but are degraded to become thermal neutrons in the tissue by collisions with hydrogen atoms. Even so, the peak in dose occurs at a depth of only 2 to 3 cm, with a rapid falloff beyond this depth. Thus, the very high surface doses are avoided, but the depth doses are still poor.
Clinical Trials
Several clinical trials have been performed over the years, beginning in the 1950s and 1960s. Results are tantalizing but never definitive. Several patients have been treated with BNCT in the United States, but the results are largely anecdotal. The concept of BNCT is as attractive as ever, but it continues to be difficult to convert to a practical treatment modality, even for shallow tumors.
▪ PROTONS
Dr. Robert Wilson first proposed protons for radiotherapy as early as 1946, based on their superior physical dose distribution; their radiobiologic properties are unremarkable. The RBE of protons is indistinguishable from that of 250-kV x-rays, which means that they are about 10% more effective than megavoltage x-rays generated by a linear accelerator. The OER for protons also is indistinguishable from that for x-rays, namely about 2.5 to 3. These biologic properties are consistent with the physical characteristics of high-energy proton beams; they are sparsely ionizing, except for a very short region at the end of the particles’ range, just before they stop. In the entrance plateau, the average LET is about 0.5 keV/µm, rising to a maximum of 100 keV/µm over a few microns as the particles come to rest. This high-LET component is restricted, however, to such a tiny length of track and represents such a small proportion of the energy deposited that, for high-energy protons, it does not have any significant effect.
To treat cancer in humans with charged particles, high-energy accelerators are necessary. Protons have been used for many years to treat choroidal melanoma of the eye as an attractive alternative to enucleation. Because the eye is small, an energy of 60-70 MeV is adequate. However, to treat deep-seated solid tumors, an energy of about 200 MeV, corresponding to a range in tissue of about 26 cm, is essential. This requires a large and expensive cyclotron or synchrotron, and so it is not surprising that the number of centers where charged particle therapy is available is limited.
Depth-Dose Patterns and the Bragg Peak
The dose deposited by a beam of monoenergetic protons increases slowly with depth, but reaches a sharp maximum near the end of the particles’ range in the Bragg peak. The beam has sharp edges, with little side-scatter, and the dose falls to zero after the Bragg peak at the end of the particles’ range. The possibility of precisely confining the high-dose region to the tumor volume and minimizing the dose to surrounding normal tissue is obviously attractive to the radiotherapist. Protons come closest to realizing this dream at modest cost.
Figure 25.2 shows the depth-dose curve for the 187-MeV proton beam from the synchrocyclotron at Uppsala, Sweden. The sharply defined Bragg peak occurs at a depth in tissue that depends on the initial energy of the particles.
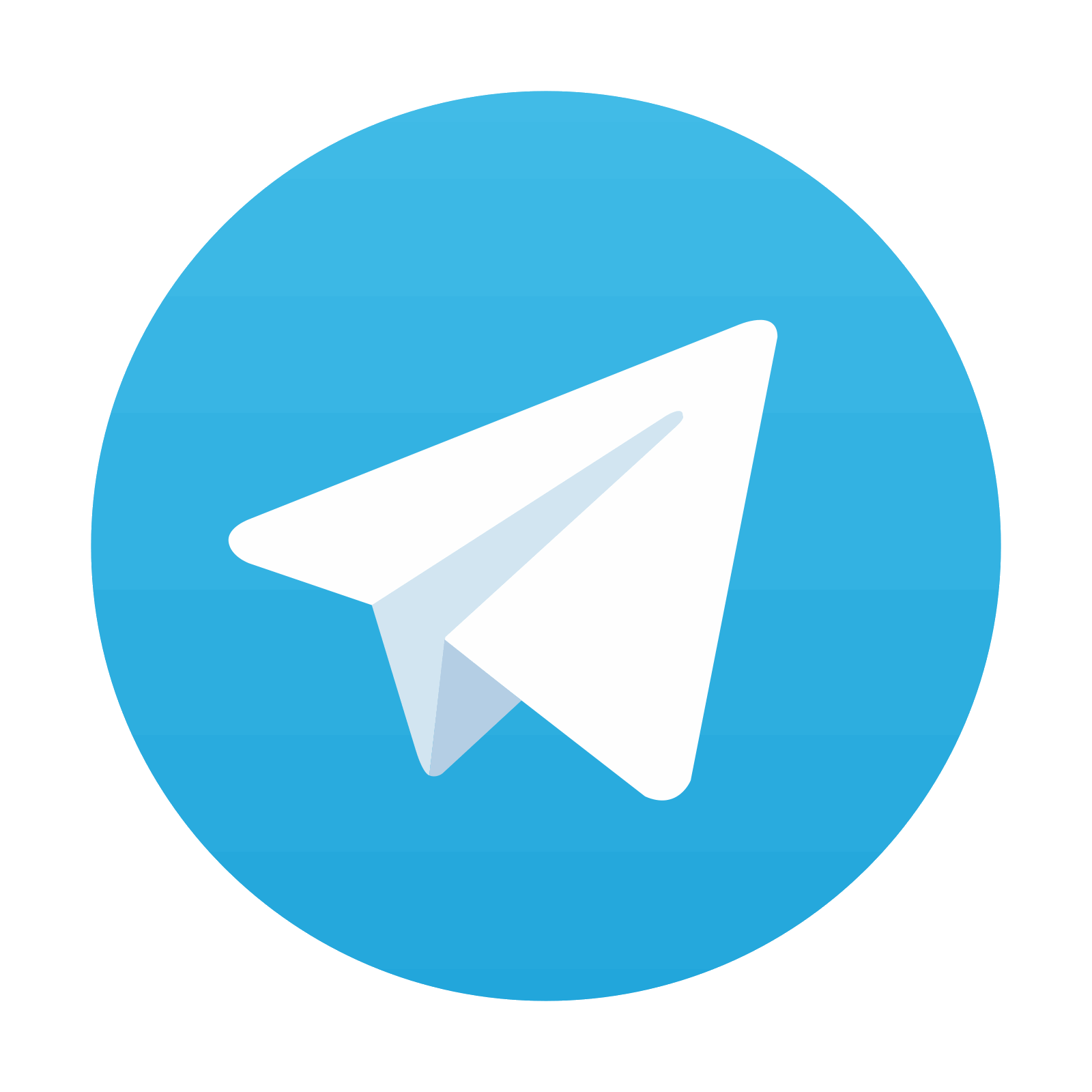
Stay updated, free articles. Join our Telegram channel
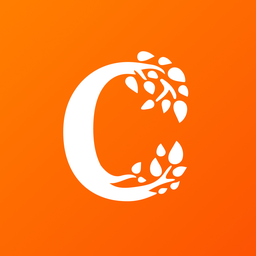
Full access? Get Clinical Tree
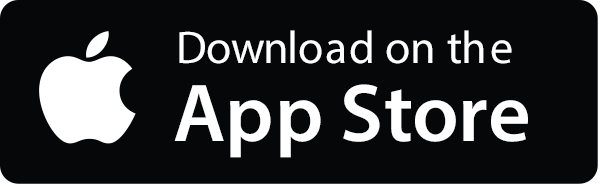
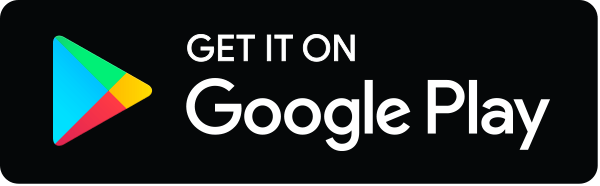