2 In Chapter 1, we discussed the physical bases of medical imaging, the atom, radioactivity, radiation, interaction of radiation with matter, and the principles of radiation detection. Medical imaging applies these processes and principles to extract biologically and clinically relevant information from the human body. Thus, a foundational discussion of medical imaging needs to include the human body. In this chapter, we first apply the principles of the interaction of radiation with matter when that interaction takes place in the context of the human body. The same principles as those of Chapter 1 apply, except the matter of interest being explicitly the human body. Medical imaging uses the transport of energy, i.e. radiation, to provide information about the subject of interest. Typically, energy is imparted to the subject, and the interaction of this energy with the biological tissue is used to understand the anatomical and physiological characteristics of the subject based on the detected energy following the interaction. Consequently, the tasks of the imaging systems are (i) to optimize the characteristics of the applied energy based on the knowledge of the interactions that may occur within the tissue/material being investigated and (ii) to match the detector system’s absorption characteristics to the radiation that is received from the patient after the interaction. Many of the characteristics of human tissue that determine the imaging results will apply to all imaging methods, but in this chapter, we will discuss primarily the attributes relevant to imaging different materials in the body using ionizing radiation. Nonionizing ultrasound (US) and magnetic resonance imaging (MRI) interactions will also be included in some sections, but more detailed discussion of the contrast mechanisms in these two modalities can be found in Chapters 9 and 10, respectively. To understand how radiation interacts with tissue, we need to understand its constituents. Water is a good analog to many soft tissues such as muscle, collagen, internal organs, and body fluids (e.g. blood and cerebrospinal fluid) as soft tissues are approximately 75% water and body fluids are 85–99% water. However, the other elements in the tissue and the structural arrangement of the molecules make the radiation interactions more complex. It is these subtle differences that are exploited in imaging studies. The elemental composition of some human tissue is shown in Table 2.1, and select physical properties that contribute to the tissues’ intrinsic imaging contrast (the intensity of the target tissue over the background intensity) are shown in Table 2.2. From these tables, it can be seen that the elemental composition of tissue and the properties that determine the attenuation of radiation do not vary greatly between most tissues. Table 2.1 Percent mass composition of tissue constituents and air Table 2.2 Properties of tissue constituents of the human body and air Plots of the mass attenuation coefficients for different tissues as a function of photon energy are shown in Figure 2.1. From these graphs, it is clear that the similarity of the mass attenuation coefficients for soft tissues, even in the lower energy range used for imaging, yields poor discrimination between most tissues, except perhaps for bone and adipose tissue. As shown in Table 2.1, bone has a larger percentage of magnesium, phosphorus, and calcium as compared to the other tissues. These higher-Z materials in bone result in an effective atomic number (Zeff) for bone almost twice that of soft tissue. This is a main reason for the difference in the mass attenuation coefficients between bone and soft tissue below 150 keV. For adipose tissue, the Zeff is about 15% less than for the other soft tissues. This is partially due to the increased carbon and decreased oxygen in adipose tissue. Although the mass attenuation coefficient for air is similar to water, it is the difference in the physical density that allows differentiation between air and tissue with radiation. Figure 2.1 Plots of mass attenuation coefficients for different tissues and air as a function of photon energy for skeletal muscle, cortical bone, adipose tissue, and dry air (a–d). The red dotted curve on each graph is the mass attenuation coefficient for water. The vertical lines indicate the energy range where most clinical imaging is performed (15–150 keV) [1]. Also seen on the plot for bone in Figure 2.1 are the absorption edges at the K-shell binding energies of phosphorous (2.1 keV) and calcium (4 keV). The K-shell binding energy for magnesium is 1.3 keV and is not seen on the graph because of its proximity to the y-axis. The K-shell binding energies from the elements that make up soft tissue are not seen on the plots because they are also at very low keV values. Regardless, because the energy values for these absorption edges are below the energy range used for imaging, most clinical applications cannot exploit the increased attenuation that occurs at the K-edge energies in biological tissues. Below about 30 keV, X-rays interact in soft tissues predominantly by photoelectric effect (see Figure 2.2). In photoelectric interactions, the photons are completely absorbed; therefore, the analysis of the number of incident to transmitted photons can be used to characterize physical properties of the objects in the beam very well. In addition, the position of the interaction within the body can be estimated precisely by the geometry of the acquisition system because the photons are not scattered when only photoelectric interactions occur. Figure 2.2 Plot of the probability of photoelectric effect vs. Compton scattering as a function of photon energy and atomic number of the material being irradiated. The gray region indicates the intersection between the range of photon energies used in clinical imaging and the range of effective atomic numbers of human tissue. Photons of higher energy interact primarily by Compton scattering with a probability that varies with the electron density of the tissue (see Chapter 1). Because photons are scattered during Compton interactions, the geometry of where the interaction occurred cannot be predicted as precisely as for the photoelectric effect. This would suggest that low-energy photons are more effective for soft tissue imaging. Although this statement makes sense from a simple interaction perspective, low-energy photons also give up a large percentage of their energy as an absorbed radiation dose to the material (e.g. a patient) (see Chapter 4). Because of this, imaging studies are always designed as a trade-off between image-quality needs, in terms of tissue penetration and differentiation, and the radiation dose to the patient. Furthermore, the actual interaction that will occur for a given photon cannot be predicted deterministically; indeed, for most imaging studies, both the photoelectric effect and Compton scattering will occur in statistical proportions dictated by the likelihood of the interaction of each photon as a function of its energy and material property. For imaging systems that do not use ionizing radiation (e.g. MR and US), different properties (namely acoustical and magnetic) of biological tissues are used to form the image, although effective atomic number, physical density, and electron density still contribute to the overall imaging characteristics. In US, the velocity of a sound wave through a material is mainly determined by the physical density of the material. The differences in ultrasonic absorption and reflection from tissues are used to form the image. In magnetic resonance (MR), the magnetic properties of hydrogen atoms are the basis of image formation, whereas the location of the molecules relative to other molecules and the energy input from the MR system contribute to the signal that can be measured. These details are discussed in Chapters 9 and 10. The principles of interactions of radiation with human tissue directly enable the body to be image-able because of the human body’s unique structures and functions. Different tissues/organs have unique appearances in the resulting images, and the practice of medical imaging is constantly working to better exploit the body’s inherent characteristics for improved extraction of relevant disease information from the body for diagnostic and therapeutic purposes. The physical characteristics of human body that are used in imaging can generally be categorized into four groups: geometrical, compositional, metabolic, and dynamic. Geometrical characteristics (i.e. anatomy) are the most obvious and widely known features of the human body. Many disease and therapy processes manifest themselves anatomically. Thus, a significant focus of medical imaging is on representing the body’s external and internal structures. Although different imaging techniques result in 2D or 3D representations of the anatomy, 3D is typically superior to 2D because it facilitates more accurate spatial renderings of overlapping structures. Depiction of the dynamic attributes of the body (e.g. cardiac and respiratory processes) is benefitted by characterizing the geometrical changes as a function of time. Although different clinical applications require different depictions of body, the primary imaging function is still to depict anatomy and allow clinicians to see spatial details inside patients in context. Tissues, objects, or features can be differentiated from one another and from the background (i.e. normal tissue in the area of interest or air outside the anatomy) based on the differences in their compositional content. This difference manifests itself in terms of contrast (a formal definition of contrast is offered in Chapter 3). In the example shown in Figure 2.3, a lung nodule looks distinct from its surround lung tissue, exhibiting a contrast with the background. Image contrast is a product of the complex interactions among the anatomic and physiologic attributes of the tissues, the characteristics of the imaging method used, the processing of the acquired data from the imaging system, and the presentation of this data as an image. Figure 2.3 Chest radiograph from a patient with lung cancer. The arrows point to two subtle lung nodules that were confirmed to be cancer. The underlying philosophy of diagnostic imaging is that structures in the patient can be distinguished in an image because they differ in physical composition and/or physiologic behavior. These differences are referred to as intrinsic (also called “subject”) contrast. For example, bone has a higher atomic number (and therefore a higher attenuation coefficient) than soft tissue, so bone will absorb and attenuate photons to a much greater extent. This provides the intrinsic contrast between the two materials, as more photons will impinge on the detector behind soft tissue than behind the bone; this difference will be depicted in an image. Some physical properties of the patient that contribute to intrinsic contrast for different imaging methods are listed in Table 2.3. Table 2.3 Physical properties of materials that influence intrinsic contrast for various imaging techniques In radiographic imaging, intrinsic contrast is mainly dependent on atomic number and physical density differences among various tissues. As seen in Tables 2.1 and 2.2, there is minimal difference in these quantities for most tissues, limiting the intrinsic contrast that can be achieved using conventional radiographic imaging. Intrinsic contrast in computed tomography (CT) depends on electron density (see Chapter 7). With CT, the subtle differences in the material properties are enhanced by calculating the linear attenuation coefficient for each type and reconstructing an image based on the calculations. Nuclear Medicine uses the distribution of a radiopharmaceutical, and the intrinsic contrast is based primarily on the activity of the radionuclide that collects in each organ (see Chapter 8). Ultrasound uses mechanical energy to propagate a sound wave into a material and analyzes the returning sound wave (see Chapter 9). The intrinsic contrast in ultrasound is based on the differences in sound propagation between materials that result in reflection, refraction, and other wave properties. In MR, there is a complex relationship between the static magnetic field and varying radiofrequency energy pulses that can be exploited to create differing image contrast for tissues (see Chapter 10). Many anatomic structures exhibit little compositional differences from one another. For example, blood vessels are visually indistinguishable from soft tissues in X-ray imaging. However, that differences can be enhanced by introducing a substance, called a contrast agent or contrast media, to alter the physical properties of structures to make them distinguishable from each other or the background. For radiographic imaging, contrast agents usually have a much higher atomic number than body tissues. The contrast materials attenuate X-rays strongly because the K-shell binding energy of these materials (e.g. K-edge of iodine = 33 keV and barium = 56 keV) is ideally positioned with respect to the energy distribution of photons typically employed for imaging. In Figure 2.4, at energies just above the K-edge, iodine in the bloodstream attenuates photons more rapidly than the surrounding tissue or the blood without iodine contrast. Figure 2.4 Mass attenuation coefficients for blood and iodine as a function of photon energy. The blue vertical lines indicate the energy range where most clinical imaging is performed (i.e. 15–150 keV). In addition to the favorable K-shell binding energy, iodine compounds are highly water soluble, and, when injected into the circulatory system, mix evenly with the blood. In this manner, blood vessels become visible in X-ray images. Imaging of vasculature with X-rays is termed angiography (see Figure 2.5). Angiography can be used to observe arterial or venous function (arteriography or venography, respectively) depending on which side of the circulatory system receives the iodine injection, and it can be used to analyze the physiology of vasculature and organ function. Figure 2.5 Angiographic images with and without contrast and following subtraction of these two images. The distribution of the contrast media is seen more clearly on the subtracted image. (a) Lateral head radiograph baseline-without contrast, (b) following contrast administration, and (c) baseline image subtracted from contrast image. Contrast agents can also be used to displace the cerebrospinal fluid in studies of the central nervous system, which is called myelography. Contrast agents are useful in CT, such as in urography studies of the kidneys and urinary tract. Compounds containing barium are frequently used for radiographic examination of the gastrointestinal tract, with the barium compound administered orally (barium swallows) or rectally (barium enemas). The solution coats the borders of the gastrointestinal tract to permit visualization of ulcers, polyps, ruptures, and other abnormalities. Compounds containing iodine or barium are relatively nontoxic and, as mentioned, may be used in a wide variety of radiographic examinations. However, some patients and some health issues (e.g. reduced kidney function) can make these agents much more toxic. The toxicity of contrast agents is dependent on two basic issues: viscosity and osmolality. Viscosity can be thought of as the thickness of the material; it can be altered by dilution, heating, and other means, but, in general, it may limit the amount of a contrast medium that can be injected at a satisfactory flow to be useful. Osmolality depends on the amount of ions produced when a substance dissolves in fluid/tissue, with contrast materials that have high osmolality termed ionic and low osmolality being nonionic. Nonionic agents are administered more frequently now due to their decreased risk of allergic reactions [2]. Air can also be used as a contrast medium in certain locations (such as the colon) where it can be introduced to displace tissues and fluids that interfere with visualization of anatomic structures of interest. As the density of air is very low, photons are transmitted through the air-filled cavities with little attenuation. Hence, the introduction of air improves the visibility of structures in or adjacent to air-filled cavities. Contrast agents are also used in US and in MR. In ultrasound, the agents generally contain microbubbles, which preferentially alter the acoustic properties of tissues. In MRI, the most common contrast agents are based on gadolinium that offers enhanced magnetic response. More details of these mechanisms are provided in Chapters 9 and 10. Intrinsic contrast, either native or enhanced with the introduction of contrast agents, need to be captured by the imaging system to be of medical use. Every imaging application reflects a choice of a specific imaging technique and image receptor to yield images with the greatest potential to demonstrate differences between different tissues, rendering the intrinsic contrast into image contrast. For a specific imaging application, the resulting image contrast can be influenced by careful selection of the technique factors (e.g. kilovolts, milliamperes) used to produce the image. In radiographic imaging, the subtle differences between the attenuation of different tissues determine the differential transmission of X-rays through the tissues. As seen in Figure 2.1, the attenuation of photons decreases rapidly as the energy of the beam increases. This implies that high-energy photons will pass though most tissue with minimal attenuation, and, therefore, the difference between tissues (i.e. intrinsic contrast) will be small. Conversely, attenuation differences between tissues are optimal at low photon energies. However, very low photon energies (less than 10 keV) are completely absorbed within most tissues through photoelectric interactions, so these energies do not contribute to image formation but only irradiate the patient resulting in an unnecessary radiation dose. Because of this, the X-ray spectrum that enters the patient should be defined based on the tissue properties and body part thicknesses under examination to ensure an optimal trade-off between the dose and the image quality. This optimization is accomplished through utilization of the appropriate X-ray tube anode material, the inherent and added beam filtration, and the technique factor used (see Chapters 1 and 6). As an example, mammography uses low-energy X-rays (between 10 and 35 keV) to optimize the intrinsic contrast between breast tissues that are similar in physical density, such as glandular and adipose tissue. Furthermore, microcalcifications in the breast can be seen because of the increased atomic number and physical density of calcium compared to glandular and adipose tissue, so higher energy X-rays could be used to visualize microcalcifications. This would allow a reduction in the patient dose without limiting the contrast. Compare this to chest radiography where relatively high peak kilovoltage (kVp) and large amounts of beam filtration are used. The higher energy X-ray spectrum produced by the higher kVp results in less attenuation of photons in bone, so they are not visualized as well (i.e. lower intrinsic contrast between tissues). This allows lung lesions to be detected, even if under the ribs (see Figure 2.3). If a lower energy X-ray spectrum was used, the attenuation in the bone would completely remove most of the photons from the beam, making the bone appear opaque and obscuring lung nodules that may lie behind them. If contrast media are used, the selection of the proper technique should be based on the optimal contrast for visualizing the contrast media, not the surrounding tissue. In Figure 2.4, photons with energy above the K-edge for iodine (e.g. just above 33 keV) have an increased attenuation. If the X-ray technique is set to a high kVp (perhaps 90–100 kVp) and the X-ray tube uses increased filtration, the X-ray spectrum produced will have its average energy just above this K-edge (see Chapter 1). On the contrary, if the technique is selected to optimize the contrast between blood and tissue without considering the use of contrast media, a much lower kVp with less filtration would be selected, and the resultant average energy spectrum would be below 33 keV. This would not optimize the attenuation of photons by the contrast media and would also result in a higher radiation dose to the patient compared to the higher energy spectrum. Living tissue is physiologically active, and disease and treatment processes can influence that activity. Many medical imaging methods aim to image body activity. Most natively, nuclear medicine (NM) is a functional modality, meaning it captures and images the metabolic characteristics of the subject as opposed to the anatomy itself. For example, NM images may display the glucose uptake of a specific region, so only the area actively using glucose will appear on the image. This mechanism leads to unique images that selectively show only the enhanced regions of the body. Currently, NM is almost always used in concert with standard anatomical imaging so that the NM-enhanced regions can be accurately identified in the patient’s body. By collecting and displaying both metabolic and geometrical/anatomical information, this hybrid imaging (such as positron emission tomography/computed tomography, PET/CT) is a powerful tool to represent a holistic fusion of the body’s functional and anatomical states (see Chapter 8). Apart from NM, MRI has also been developed into imaging metabolic activities through functional MRI and spectroscopy. US and CT are likewise being explored for metabolic explorations through the advent of targeted contrast agents with functional accumulation properties. Living tissue is not inert: patients themselves can move during an imaging study, and their internal organs are in continuous flux because of blood flowing, digestion, breathing, etc. Faster imaging techniques, such as one-shot radiographs, are the best modalities in terms of limiting voluntary or involuntary patient motion. Generally speaking, radiography (including mammography and fluoroscopy) is better than CT, which is better than US, MR, and NM imaging to freeze the body motion. However, sometimes, the primary goal of a study can be capturing movement (such as blood velocity or cardiac motion). Voluntary or involuntary motion is often problematic in imaging studies because they blur the anatomy being imaged. However, motion can also be used to characterize the physiological function, the tissue’s environment, or the physical state of the tissue. The movement of a material only occurs through the application of a force. At the atomic level, the spin rate (i.e. magnetic moment) of the nuclei responds to the force from an external magnetic field, and how these atoms and molecules interact and react to the surrounding environment can be exploited to measure the physical states with MR imaging and spectroscopy. At the molecular level, varying concentration of a material or the electrical potential between regions can provide the force to produce motion across cell membranes and within tissues (e.g. diffusion). At larger scales, changes from tissue rigidity and fluid pressure within a region may be the primary force that determines the motion (e.g. elastography). Regardless of the scale, analyzing the motion of a material requires an understanding of mechanics, thermodynamics, and fluid dynamics (i.e. kinetics of the material and its environment) to grasp how an imaging protocol can be used to acquire information about the physiology. Kinetics in imaging often refers to processes of fluid perfusion (e.g. blood) in human tissue. Perfusion, which is simply the passage of fluid into an organ/tissue, is influenced by heat and momentum, which transports energy and mass through the body (predominately through diffusion mechanisms). The mathematics of these transport methods for many biological systems were originally derived by Adolf Fick (i.e. the Fick principle or Fick’s law of diffusion) [3–5]. Most molecules move randomly, but when there are a large number of molecules, Fick’s law can be used to describe processes such as flux, diffusion, and concentration; the law essentially calculates the rate of particles moving across a surface as where P is the pressure (or partial pressure if interested in only certain molecules), A is the surface area, t is the time, and D is the diffusion constant (solubility/square root of molecular weight ratio). Similarly, Graham’s law (a rearrangement of Fick’s law) delineates the relationship between the net rate of particles moving through an area (flux) and the gradient, which is the change in pressure (particles in a volume) over a distance: Henry’s law defines the concentration of molecules, showing that the amount of dissolved gas is proportional to its (partial) pressure in the gas phase as where KH is a constant based on temperature and the materials in different regions. The above laws govern the perfusion processes through the human tissue. However, apart from perfusion, there are a host of other kinetic attributes in the human body that lend themselves to imaging interrogations. They include stress and strain, diffusion, laminar and turbulent flow, compressibility, viscosity, and pressure. As with perfusion, many of these methods are being used clinical in imaging studies and ongoing research is exploring these and other physiological mechanisms to further enhance how they can be used, although detailed analysis of these processes and uses are beyond the scope of this text. For understanding medical imaging, it is important to have a basic understanding of normal anatomy and physiology, how they are altered by disease (pathology), and how imaging plays a role in the diagnosis and management of the disease. This section summarizes those aspects of anatomy, physiology, and pathology that are pervasive in medical imaging, and how the major imaging modalities aid in the diagnosis and patient management. Physicians have developed terminology to precisely describe how the easily visible parts of the body relate to each other and to the observer. The body may be viewed from four aspects: anterior, posterior, medial, and lateral (identifying both body surfaces and the positions of structures relative to each other), and in four directions: right, left, superior, and inferior (Figure 2.6). Although these descriptions are sufficient for planar radiography, cross-sectional imaging (CT or MRI) requires the use of planes and a three-dimensional coordinate system to fully describe the locations and directions within the body (Figure 2.7). Figure 2.6 Nomenclature for imaging directions. The terms “rostral” and “caudal” may be substituted for “superior” and “inferior,” respectively, and “ventral” and “dorsal” for “anterior” and “posterior,” respectively. Figure 2.7 Nomenclature for imaging planes. The terms “frontal” may be substituted for “coronal” and “transaxial” for “transverse.” The sagittal plane that bisects the body into symmetric right and left halves is called the “midsagittal” plane. The primary body axis, or z-axis, is a line extending from the pelvis through the center of the body to the top of the head. A transaxial, usually called axial, or transverse plane is a plane located along and perpendicular to the z-axis. A coronal, or frontal, plane is parallel to the z-axis and extends in the left–right direction. A sagittal plane is parallel to the z-axis and extends in the anterior–posterior direction. The sagittal plane that bisects the body into left and right halves is called the midsagittal plane. The x– and y-axes lie in the transaxial plane. Although image display systems permit the operator to view any arbitrary plane, the transaxial, coronal, and sagittal planes are routinely used in visualizing anatomy and describing findings. It is critically important that images be viewed using standard aspects; doing otherwise has led to disastrous results. For example, a planar radiograph of the lower extremities that is viewed by a surgeon who unwittingly reverses right and left has led to the amputation of the wrong leg. To prevent this type of error, standard display orientations are employed for all imaging modalities. For interventional procedures, such as needle biopsies, changes in the patient’s position on the table may lead to deviations from the standard display orientations, and care must be taken when interpreting these images. Classically, the study of anatomy began at the head and proceeded down the body to the feet, but physiology was studied differently. Because function is so intimately related to structure, an alternative organ-system-based method has supplanted the classical method recognizing the body into nine major organ systems (Figure 2.8). Using this approach, the anatomy, physiology, and pathology of an organ system can be fully integrated with the imaging methodologies employed in diagnosis. Figure 2.8 The structure of human body’s nine major body systems.
Anatomy, Physiology, and Pathology in Imaging
2.1 Introduction
2.2 Interaction of Radiation with Tissue
2.2.1 Properties of Human Tissue
Element (Z)
Adipose tissue (i.e. fat)
Muscle
Water
Bone
Air
Hydrogen (1)
11.2
10.2
11.2
8.4
Carbon (6)
57.3
12.3
27.6
Nitrogen (7)
1.1
3.5
2.7
0.78
Oxygen (8)
30.3
72.9
88.8
41.0
0.21
Sodium (11)
0.08
Magnesium (12)
0.02
7.0
Phosphorus (15)
0.2
7.0
Sulfur (16)
0.06
0.5
0.2
Potassium (19)
0.3
Calcium (20)
0.007
14.7
Material
Adipose tissue (i.e. fat)
Muscle
Water
Bone
Air
Effective atomic number (Zeff)
5.9–6.3
7.4
7.4
11.6–13.8
7.6
Physical density (kg/m3)
0.91
1.00
1.05
1.65–1.85
0.001 29
Electron density (×1026 electrons/kg)
3.34–3.48
3.36
3.34
3.00–3.10
3.01
2.2.2 Human Body Attributes Influencing Imaging
2.2.2.1 Geometrical Characteristics
2.2.2.2 Compositional Characteristics
2.2.2.2.1 Intrinsic Differences
Radiography and fluoroscopy
Computed tomography
Nuclear medicine
Ultrasound
Magnetic resonance
Atomic number
Electron density
Radiopharmaceutical activity
Compressibility
Element species density (e.g. proton)
Physical density and thickness
Physical density and thickness
Radiopharmaceutical distribution
Physical density and thickness
Molecular binding characteristics
Flow
Flow
Flow
Motion and flow
Motion and flow
2.2.2.2.2 Contrast Agents
2.2.2.2.3 Captured Differences
2.2.2.3 Metabolic Characteristics
2.2.2.4 Dynamic Characteristics
2.2.2.4.1 Motion
2.2.2.4.2 Kinetics
2.3 Structure and Function
2.3.1 Imaging Planes, Directions, and Standard Display
2.3.2 Organ Systems, Pathology, and Depiction
2.3.2.1 Skeletal System
2.3.2.1.1 Skeletal Structures and Functions
Stay updated, free articles. Join our Telegram channel
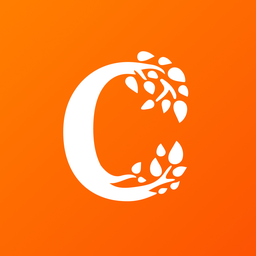
Full access? Get Clinical Tree
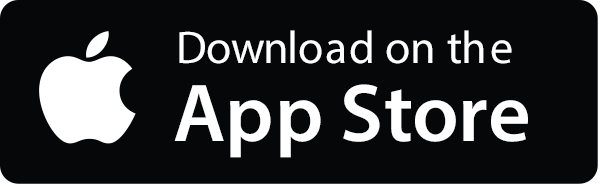
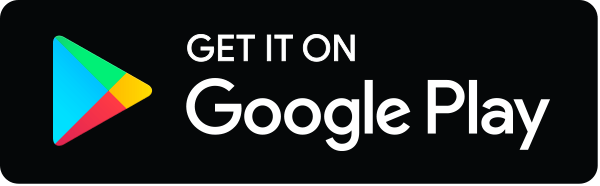