CHAPTER 23 Aneurysms
Aneurysms are focal abnormal dilatations of an artery. In 1997, Schievink proposed that “intracranial arterial aneurysms are acquired lesions that are most commonly located at the branching points of the major cerebral arteries coursing through the subarachnoid space at the base of the brain.” He pointed out that “intracranial arteries have an attenuated tunica media and a lack of external elastica lamina” and that “intracranial arterial aneurysms have a thin tunica media or none, and the internal elastica lamina is either absent or severely fragmented.” These observations are generally correct but do not attempt to distinguish among the diverse diseases that give rise to arterial aneurysms. The concept that the arterial bloodstream first “expands” and then “bursts” an “aneurysmal herniation of the wall” is now considered too simple to explain the complex features of arterial aneurysms. Different classification schemes have been based on aneurysm size (small vs. large vs. giant), location (posterior circulation vs. anterior circulation), clinical presentation (ruptured vs. unruptured), morphology (saccular vs. fusiform), or etiology (false or traumatic aneurysms, dissecting aneurysms, flow-related aneurysms, infectious aneurysms). Each of these classifications has advantages, but we will use the etiologic classification in this chapter because therapeutic decision-making can be improved by a better understanding and recognition of the many different lesions grouped together as aneurysms (Fig. 23-1).
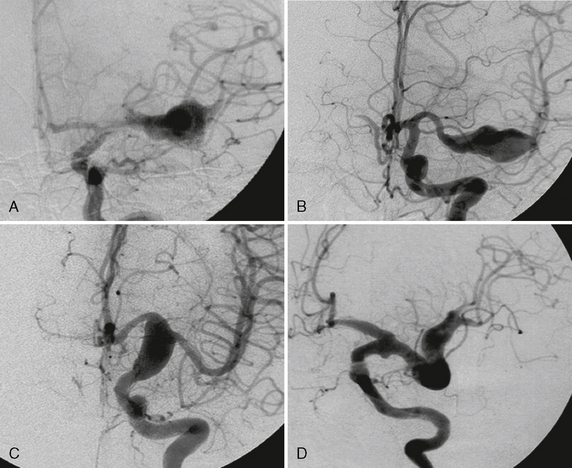
FIGURE 23-1 Same morphologic type of aneurysm (fusiform aneurysm) depicted in four different patients. The term fusiform aneurysm corresponds to an enlargement of the entire vessel circumference over a segment of the artery and is a morphologic description rather than a pathomechanism. Although these aneurysms look similar, their pathomechanisms are completely different, inferring different treatment strategies. The four different underlying etiologies are aneurysm in the presence of atrial myxoma most likely resembling a neoplastic aneurysm (A), acute transmural dissection with subarachnoid hemorrhage (B), aneurysmal dilatation present in familial candidasis (C), and fusiform lumen of a partially thrombosed aneurysm following a healed dissecting process (D).
EPIDEMIOLOGY
Mirror-Image or Twin Aneurysms
A special subgroup of multiple aneurysms is the “mirror-like” or “twin” aneurysms at symmetric sites on each side. Twin aneurysms occur in 5% to 10% of all aneurysm patients but in as many as 36% of all multiple aneurysm patients. They are found on all intracranial vessels but are most common in the MCA. Twin aneurysms may be discovered in the same clinical context as “classic” saccular aneurysms (e.g., during SAH), but their origin is likely to be different. The occurrence of mirror-like aneurysms, their association with familial disease, and their tendency to rupture earlier in life than “classic” aneurysms suggests that congenital weakness of the vessel wall may be an underlying cause (Fig. 23-2).
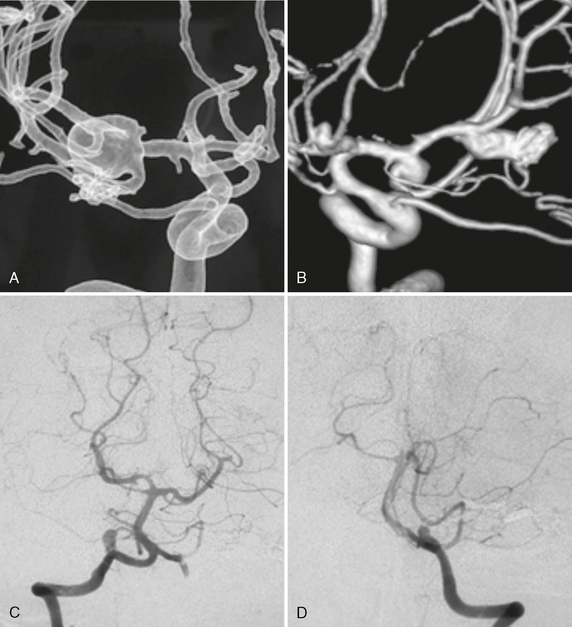
FIGURE 23-2 A to D, Multiple mirror aneurysms in a single patient who harbored bilateral symmetric MCA aneurysms, bilateral symmetric PICA aneurysms, and an anterior communicating artery aneurysm. The multiplicity and the mirror or twin occurrence suggest a congenital predisposition to be more likely than degenerative causes alone for the appearance of these aneurysms.
Familial Forms and Screening
Intracranial aneurysms are found approximately twice as often in first-degree relatives of patients with aneurysmal SAH than in the general population. Within families, the greater the number of members affected, the greater the risk that any one member will have an aneurysm, up to four times the incidence in the general population. Females predominate (54% to 70%) in familial SAH. In males, the SAH occurs 7 to 10 years earlier in familial forms of aneurysms than in nonfamilial forms. Sibling pairs with arterial aneurysms tend to bleed within the same decade. Most familial arterial aneurysms arise in the MCA territory (50%), especially on the right side, and tend to bleed at relatively small arterial aneurysm size (<6 mm at rupture). The rate of multiplicity is not higher in familial arterial aneurysms, but collected data suggest that fewer arterial aneurysms remain asymptomatic in SAH families.
SACCULAR ANEURYSMS
Aging, hypertension, and/or smoking are thought to lead to general thickening of the intima of arteries, which is most pronounced distal and proximal to branching sites. These thickened intimal “pads” are inelastic and lead to an increased strain on more elastic parts of the vessel wall adjacent to these pads. Increased strain on the vessel leads to an increased activity of metalloproteinases and other extracellular matrix-degrading proteins, culminating in aneurysmal outpouching of the vessel wall. A vicious circle may then ensue as turbulent flow against the outpouching increases the strain on the vessel wall. Such hemodynamic stress may be especially significant at sites of congenital weakness of the vessel wall. For example, aneurysms often form in the vicinity of anatomic variations such as incomplete fusion of the basilar artery or unilateral absence of the horizontal segment of an anterior cerebral artery (A1) (Fig. 23-3). However, these theories are not able to explain why certain patients develop aneurysms and others with similar “offensive exposures” do not. Therefore, individual defects in host response (e.g., a missing defensive line) may be present that finally lead to the formation of an aneurysm.
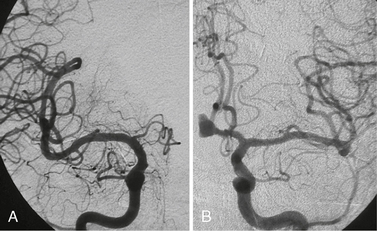
FIGURE 23-3 A and B, In the absence of a contralateral A1 segment, aneurysms tend to form more often on the ipsilateral anterior communicating ramus. Whether this is due to increased hemodynamic shear stress forces or to a relative immaturity (and therefore increased fragility) of the vascular system is unclear.
The construction and maintenance of blood vessels are the result of complex biologic factors and events that involve repetitive steps and feedback to the vascular tree. The structural integrity of arteries is maintained and modified according to hemodynamic or metabolic demands (e.g., shear stress forces), which are genetically programmed and controlled. Vascular remodeling is an active and adaptive process of structural alteration and includes changes in cellular processes such as cell growth, cell death, cell migration, and production or degradation of the extracellular matrix. Shear stress forces activate specific (genetically programmed) steps that alter the balance of the mediators of remodeling (e.g., metalloproteinases, nitric oxide synthase, platelet-derived growth factor [PDGF], and transforming growth factor β1). Aging decreases the capacity of the vessel wall to adapt to variations in the hemodynamic parameters or to compensate for stressful events. This progressive aging process is an acquired vulnerability of the vessel wall, which will differ in males and females and with patient age. Congenital alterations in these programs could well result in a variant, defective, or absent reconstruction of the vessel wall. Similarly, we believe that the association of arterial variants with arterial aneurysms points to an incomplete maturation process of the arterial wall. The lack of cell selection that such a pattern implies may preserve “weaker,” that is, less mature endothelial cells that will later develop arterial aneurysms when subject to secondary triggers such as hemodynamic stress.
In the individual patient there is a complex interplay between the aggressive “offensive” factors and the host response. Formation of an aneurysm, therefore, indicates failure of the repair system at a given period in time and/or the persistence of the abnormal triggering factors responsible for the failure of the remodeling processes. Therapy can then be targeted either toward augmenting the host’s defensive system (not yet possible) or toward ameliorating local, aggressive “offensive” factors leading to the aneurysm. The ultimate goal is to stimulate the vascular remodeling system to repair the local injury to the vessel wall triggered by the offensive insult. Spontaneous resolution of berry-like aneurysms, seen after correction of abnormal flow dynamics, holds the promise of such therapy in the future (Fig. 23-4).
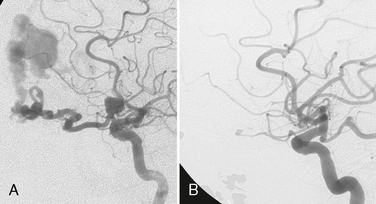
FIGURE 23-4 A and B, Spontaneous resolution of an ophthalmic artery aneurysm that was associated with a high-flow shunting lesion of the ophthalmic artery that spontaneously regressed after occlusion of the shunt. This example testifies for the repair system of the vascular system after removing locally aggressive factors.
Clinical Presentation
Most saccular aneurysms of the intracranial circulation remain undetected until they rupture and present as SAH. Arterial aneurysms are the most important cause of primary nontraumatic SAH and account for 65% to 85% of all SAH (Fig. 23-5). After acute SAH, recurrent hemorrhage from the aneurysm poses continued serious threat to the patient, with an estimated risk of re-rupture of more than 20% at 2 weeks and 40% at 6 months. Therefore, treatment of ruptured aneurysms is mandatory.
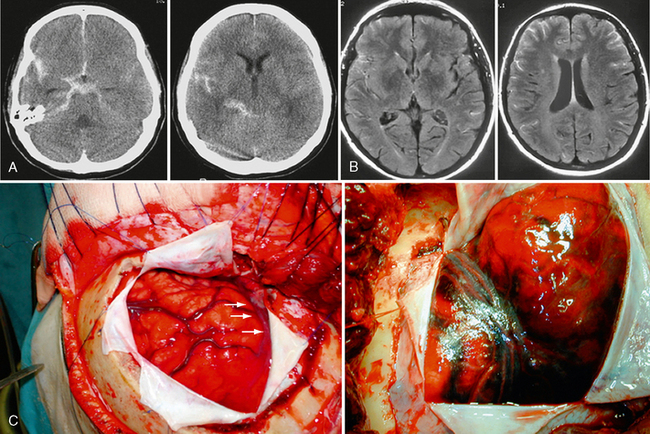
FIGURE 23-5 Subarachnoid hemorrhage. A, On cranial CT, a subarachnoid hemorrhage is typically hyperdense and fills the subarachnoid spaces. B, On FLAIR-weighted images, blood in the subarachnoid space fails to suppress like usual CSF and is therefore bright as well. The distribution of blood may shed light on the location of the aneurysm, which may be of special importance in the presence of multiple aneurysms. C, Intraoperative photos following opening of the dura, with extensive blood in the subarachnoid space and brain swelling (“angry brain”).
The clinical consequences of SAH are so severe that emphasis should be placed on the prevention of rupture once the aneurysms are detected incidentally. Therefore, it is useful to identify factors that stabilize or destabilize the aneurysm. Factors known to predict increased risk of future hemorrhage include prior SAH from a different aneurysm, large aneurysm size, and location in the posterior circulation, as shown by the International Study of Unruptured Intracranial Aneurysms (ISUIA) study (Table 23-1). Additional factors suggesting increased risk of rupture include familial SAH, smoking, specific aneurysm morphology (e.g., multilobulated berry aneurysms with “daughter” aneurysms or aneurysmal “blebs”), multiplicity of aneurysms, and the presence of arterial variations in the vicinity of an aneurysm (pointing toward an incomplete maturation that may preserve “weaker” cells that may be more prone to rupture).
TABLE 23-1 Five-Year Cumulative Percentage Risk of Rupture of Previously Unruptured Aneurysms According to the ISUIA Study

Imaging
CT and CTA
Multislice CT (MSCT) is a technique in which scanning is performed continuously as the CT table is drawn through the gantry (helical or spiral scanning). At least two rows of detector elements (and, at present, as many as 320 rows of detector elements) are employed in the z-axis to create almost isotropic voxels. Although there is no standard protocol, the technique typically entails injection of high-concentration (300 to 400 mg/mL) iodinated contrast media at a dose of 1 to 2 mL/kg. Injection is made through an antecubital vein at a flow of 3 to 5 mL/s up to a total of 60 to 100 mL. The scanned region extends from the C1 vertebra to the vertex. What slice thickness is possible depends on scanner performance (Fig. 23-6). Generally, slice thickness ranges from 0.5 to 1.25 mm, with a reconstruction interval of up to 0.6 mm. In many centers, a scan is started using a triggering technique to optimize acquisition of early arterial phase images. After real-time CT bolus tracking, the region of interest is placed at the internal carotid artery, and scanning is started automatically when the contrast agent in the ICA reaches 80 Hounsfield units (HU). With the use of triggering and real-time CT the increased attenuation of acute subarachnoid blood has no effect on the ability to define the vessels during 3D reconstruction, because it remains lower than the attenuation of the enhanced vessels in all cases. With this technique even small vascular structures can be imaged. The CT data can then be reconstructed to produce maximum intensity projection (MIP) or 3D representations employing shaded surface displays (SSD) or volume rendering techniques (VRT). The main drawback of the MIP images is vessel superposition, which may impair appreciation of vessel relationships. Multiplanar reconstructions of variable thickness may overcome superposition problems. The VRT allows direct 3D analysis; however, bone or venous superposition in the cavernous sinus region may render carotid siphon or posterior communicating artery aneurysms difficult to appreciate. Compared with single-slice CT, the concurrent acquisition of multiple slices and the superior resolution in z-axis in MSCT allows for a dramatic reduction of scanning time and an improvement of visualization of aneurysms.
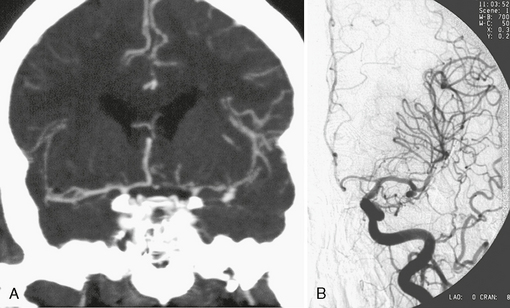
FIGURE 23-6 CTA (A) and DSA (B) in the detection of a small MCA aneurysm. The major advantages of CTA over other angiographic methods is its added information about the surrounding bony structures, the possible detection of associated calcifications close to the aneurysm neck, the depiction of extraluminal parts of the aneurysms (such as present in partially thrombosed aneurysms), and the accurate 3D demonstration of the aneurysm and its neck. Because CTA is widely available and CT is the first diagnostic choice to detect subarachnoid hemorrhage, many centers perform CTA immediately after CT detection of a subarachnoid hemorrhage for subsequent treatment planning.
MRI and MRA
MR pulse sequences can exploit blood motion to visualize vascular structures directly and without the use of intravascular contrast material. However, MRI and MR angiography (MRA) are seldom used in the setting of acutely ruptured aneurysms because patient monitoring may be difficult, imaging time is typically more extended compared with CT, the field of view is often limited, and specific MR artifacts (discussed later) are likely to result in a lower sensitivity and specificity of aneurysm detection. Although T2-weighted (T2W) fluid-attenuated inversion recovery (FLAIR) sequences are supposed to detect SAH (see Fig. 23-5), artifacts in the perimesencephalic cistern are often present that make identification of true SAH difficult. Nonetheless, MRA may still be useful for screening and follow-up of previously detected aneurysms, because it is noninvasive, uses no ionizing radiation, and requires no iodinated contrast media.
Many of these problems can be overcome with CE MRA, which is performed in a manner analogous to conventional contrast angiography. Instead of relying on blood motion to create intravascular signal, a contrast agent is introduced to change the T1 relaxation of blood. Blood can be directly imaged regardless of flow, which alleviates many of the problems inherent to TOF and PC angiography. Contrast medium can reduce spin saturation due to its T1 shortening effect. Shorter echo time then diminishes phase dispersion. Therefore, sensitivity to turbulence is dramatically reduced and in-plane saturation effects are eliminated. The technique allows a small number of slices oriented in the plane of the vessels of interest to image an extensive region of vascular anatomy (equal to the field of view) in a short period of time. At present the major shortcoming of CE MRA is its limited spatial resolution. Small aneurysms are likely to remain undetected using this technique. With the different MR angiographies, sensitivities and specificities of 50% to 100% have been reported depending on the cited source, the technique used, the reconstruction algorithm used, and the employed field strength.
Differential Diagnosis
Aneurysm Distribution
Aneurysms typically arise at branch points on the parent artery. The branch point may be formed by the origin of a side branch from the parent artery (e.g., posterior communicating artery) or by subdivision of a main arterial trunk into two trunks (e.g., MCA or terminal ICA bifurcation, tip of the basilar trunk). Sidewall aneurysms are rare and if present usually arise at a turn or curve in the artery and point in the direction that the blood would have gone if the curve was not present. At both the branch points and the curves, local alterations in intravascular hemodynamics are present that exert high shear stress forces on those regions that receive the greatest force of the pulse wave. Therefore, the aneurysm dome typically points in the direction of the maximal hemodynamic thrust in the pre-aneurysmal segment of the parent artery. Aneurysms that are encountered on a straight, nonbranching segment of an intracranial artery should raise the suspicion of a dissecting process, because saccular aneurysms are infrequently encountered at these sites. More than 90% of all saccular intracranial aneurysms are located at one of the following five sites:
• Internal carotid artery at the origin of the posterior communicating artery
• Junction of the anterior cerebral artery (ACA) and anterior communicating artery
• Proximal bifurcation of the MCA
• Terminal bifurcation of the basilar artery into posterior cerebral arteries
• Terminal bifurcation of the carotid artery into the ACA and MCA
Supraclinoid Segment of the Internal Carotid Artery
• The upper surface of the ICA at the origin of the ophthalmic artery (supraophthalmic aneurysms) (∼5%)
• The medial wall of the ICA at the origin of the superior hypophysial artery (hypophysial artery aneurysms) (∼1%)
• The posterior wall of the ICA superolateral to the origin of the posterior communicating artery (posterior communicating aneurysms) (∼25%)
• The posterior wall of the ICA immediately superior to the origin of the anterior choroidal artery (anterior choroidal artery aneurysms) (∼5%)
• The apex of the terminal ICA bifurcation into the ACA and MCA (carotid bifurcation aneurysms) (∼5%)
Supraophthalmic Artery and Superior Hypophysial Artery Aneurysms
Aneurysms arising from the ICA close to the ophthalmic artery constitute about 5% of all intracranial aneurysms and are more frequent in women than men. They typically arise from the superior wall of the carotid artery at the distal edge of the origin of the ophthalmic artery close to the roof of the cavernous sinus (Figs. 23-7 to 23-9). At this point, the ICA changes direction from superior toward posterior, so the maximal hemodynamic force is directed toward the superior wall of the carotid artery just distal to the ophthalmic artery. Therefore, these aneurysms project upward toward the optic nerve. Supraophthalmic aneurysms are easily approached endovascularly but may be complicated to expose surgically, because the ophthalmic artery has a variable origin and course and because multiple folds of the dura enclose the region of the optic foramen and clinoid process. Supraophthalmic aneurysms are often large with complex, multilobulated shape. Many are wide-necked aneurysms that may require remodeling techniques. Unruptured aneurysms may become symptomatic due to headaches or compression of cranial nerves.
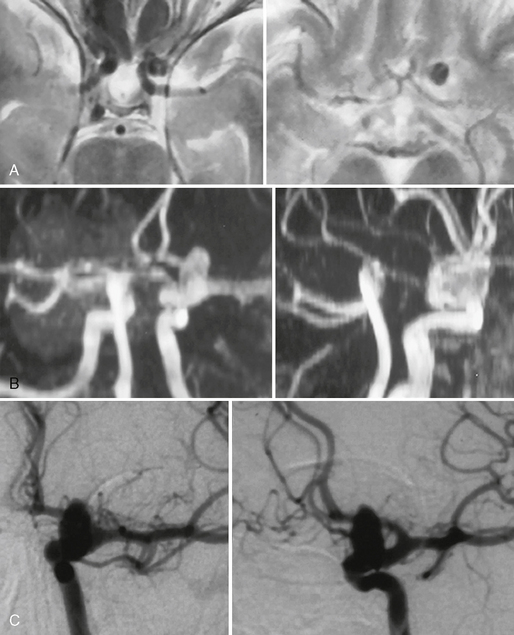
FIGURE 23-7 Internal carotid artery aneurysm at the origin of the supraophthalmic artery (“ophthalmic aneurysm”) that was incidentally found in a patient who was evaluated for headaches. A, T2W MR sequences demonstrate a flow void that is directed into the subarachnoid space. B, TOF MRA is able to demonstrate the origin of the aneurysm that was verified by DSA (C).
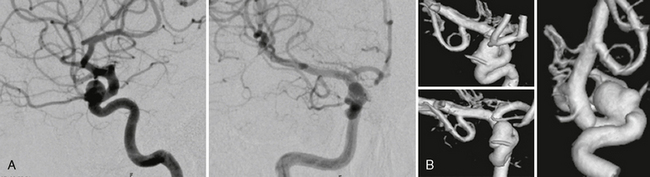
FIGURE 23-8 A, Supraophthalmic ICA aneurysms are often broad based and typically large. The origin of the ophthalmic artery has to be specially considered to avoid erratic emboli entering the artery during endovascular therapies. B, Most often, 3D rotational angiography is necessary to determine the exact localization of the artery in relation to the neck of the aneurysm.
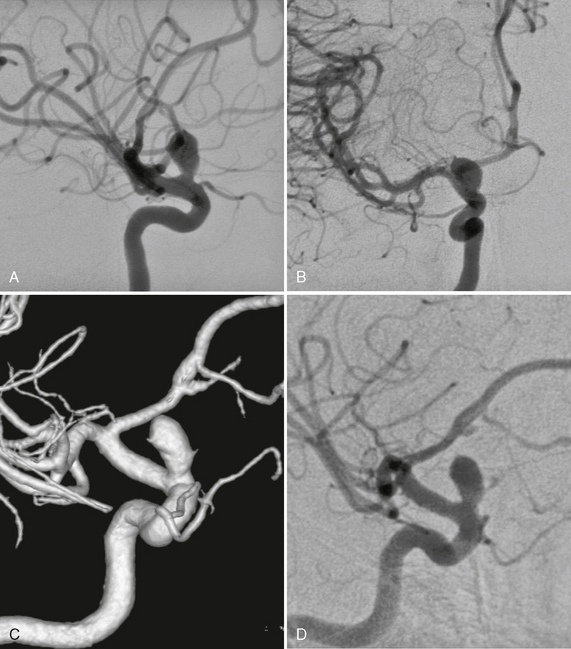
FIGURE 23-9 A to D, Small-necked large aneurysm of the ICA at the origin of the ophthalmic artery. Typically these aneurysms point superiorly owing to the hemodynamic thrust in this vessel segment.
Superior hypophysial artery aneurysms arise just distal to the origin of the superior hypophysial artery from the medial or posterior wall of the ICA where the curvature of the ICA is convex medially (Fig. 23-10). In this location they lie lateral to the pituitary stalk and point medially under the optic chiasm. Medial expansion of the aneurysm may compromise the perforating arteries to the floor of the third ventricle, the optic nerves, the chiasm, the pituitary stalk, and the hypophysial vascular supply.
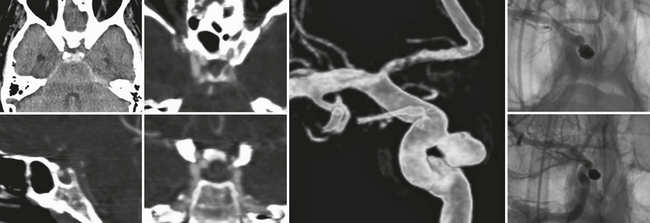
FIGURE 23-10 Aneurysm of the internal carotid artery that is directed medially into the superior part of the sella and most closely resembles a superior hypophysial artery aneurysm that in this patient led to a circumscribed subarachnoid hemorrhage, because the superior aspect of the aneurysm is not completely extradural but points toward the dural fold surrounding the cavernous sinus. CTA is especially helpful to detect the close spatial relationship between the aneurysm and the bone, whereas 3D rotational angiography can plan the subsequent endovascular treatment that was carried out with a complete and dense coil packing in this patient.
Posterior Communicating and Anterior Choroidal Artery Aneurysms
The posterior communicating and anterior choroidal arteries arise from the posterior wall of the ICA, where the ICA forms a posteriorly convex curve as it ascends to its terminal bifurcation under the anterior perforated substance. The most frequent ICA aneurysm is the posterior communicating artery aneurysm, which constitutes about 25% of all intracranial aneurysms (Figs. 23-11 to 23-14). These aneurysms arise near the apex of the posteriorly convex turn, immediately superior to the distal edge of the origin of the posterior communicating artery. They point downward and posteriorly toward the oculomotor nerve, so the posterior communicating artery is usually found inferomedial to the neck of the aneurysm and the anterior choroidal artery is found superior or superolateral to the neck of the aneurysm. The oculomotor nerve enters the dural roof of the cavernous sinus lateral to the posterior clinoid process and medial to a dural band that runs between the tentorium cerebelli and the anterior clinoid process. Posterior communicating artery aneurysms larger than 4 to 5 mm may compress the oculomotor nerve at its entrance into the dural roof, causing ophthalmoplegia.
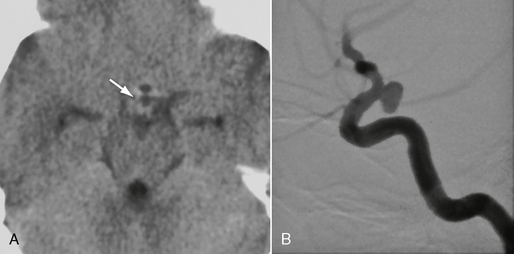
FIGURE 23-11 This patient had a sudden oculomotor nerve palsy on the right side. A, Nonenhanced CTA demonstrated a dense structure within the subarachnoid spaces within the pentagon of the basal cisterns (arrow). Because of the clinical presentation and this suspicious CT finding, DSA was performed that demonstrated a typical posterior communicating artery aneurysm (B), that, owing to its downward and posterior direction, had led to irritation of the third cranial nerve.
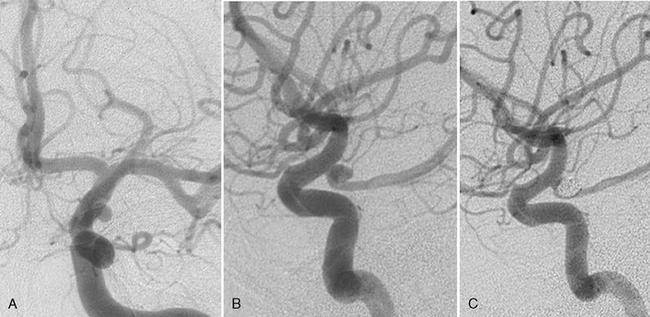
FIGURE 23-12 A to C, Classic posterior communicating artery aneurysm that originated above the distal edge of the origin of the posterior communicating artery that is found inferomedial to the aneurysm. Endovascular therapy was performed that resulted in complete occlusion of the aneurysm with preservation of the parent artery.
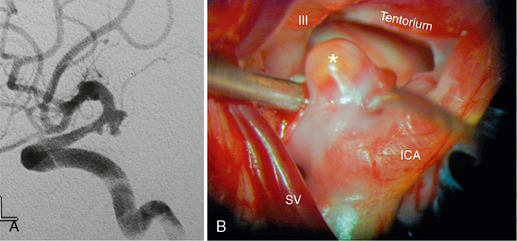
FIGURE 23-13 DSA (A) and corresponding surgical aspect (B) of a posterior communicating artery aneurysm (asterisk) and its close spatial relationship to the oculomotor nerve (III) and the tentorium cerebelli. ICA, internal carotid artery; SV, sylvian vein.
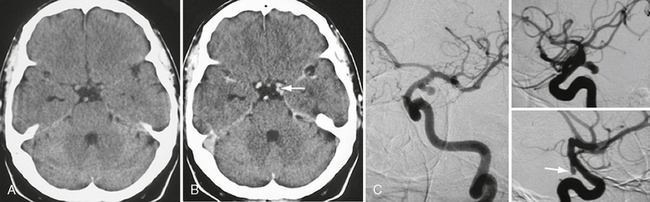
FIGURE 23-14 Unenhanced (A) and enhanced (B) cranial CT images in a patient with a spontaneous oculomotor nerve palsy on the left side demonstrating an “additional” vessel within the pentagon of the basal cisterns (arrow in B) that was found to be a classic posterior communicating artery aneurysm on DSA (C
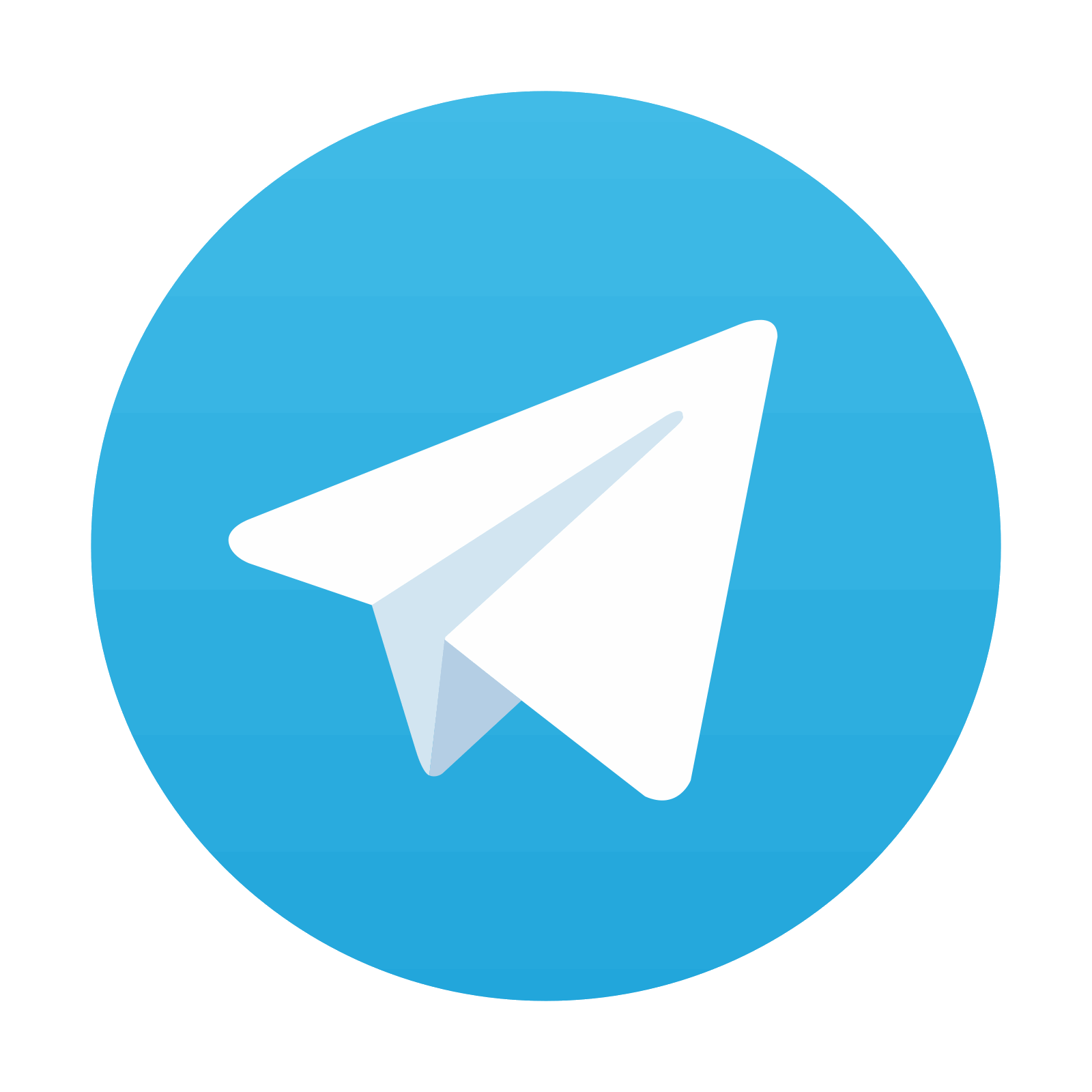
Stay updated, free articles. Join our Telegram channel
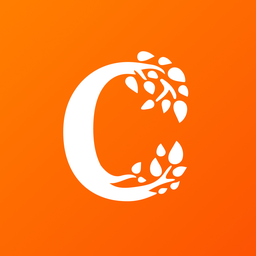
Full access? Get Clinical Tree
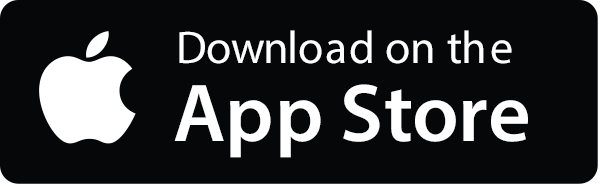
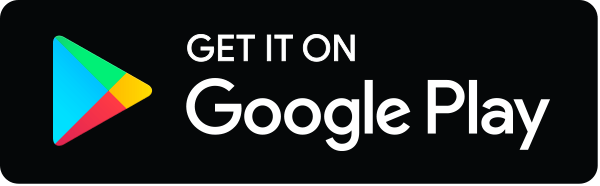
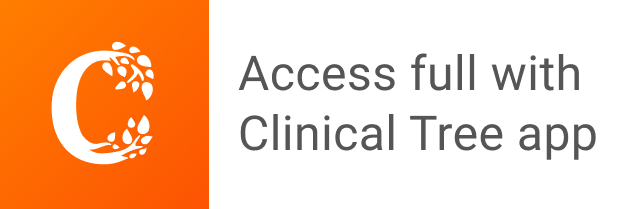