(1)
Department of Nuclear Medicine, Kuwait University, Safat, Kuwait
14.1 Radionuclide Therapy
14.2.1 Pathophysiology
14.4.1 Radiopharmaceuticals
14.4.2 Mechanism of Action
14.4.4 Clinical Use
14.6 Radioimmunotherapy
14.7.2 Mechanism of Action
14.7.4 Clinical Use
Abstract
Therapeutic applications of nuclear medicine are expanding (Table 14.1). The use of radioisotopes in therapy was limited predominantly to treatment of hyperthyroidism, thyroid cancer, and polycythemia rubra vera. Strontium-89 (89Sr), rhenium-186 (186Re), samarium-153 (153Sm), and tin-117m (117Sn) have been increasingly used recently in treating bone pain secondary to metastases. Additionally, treatment of certain neuroendocrine tumors with 131I-MIBG and labeled octreotide and pentreotide, the use of radiolabeled monoclonal antibodies for lymphomas, radionuclide synovectomy, and treatment of hepatic primary and metastatic lesions have revolutionized the field of therapeutic nuclear medicine.
14.1 Radionuclide Therapy
Therapeutic applications of nuclear medicine are expanding (Table 14.1). The use of radioisotopes in therapy was limited predominantly to treatment of hyperthyroidism, thyroid cancer, and polycythemia rubra vera. Strontium-89 (89Sr), rhenium-186 (186Re), samarium-153 (153Sm), and tin-117m (117Sn) have been increasingly used recently in treating bone pain secondary to metastases. Additionally, treatment of certain neuroendocrine tumors with 131I-MIBG and labeled octreotide and pentreotide, the use of radiolabeled monoclonal antibodies for lymphomas, radionuclide synovectomy, and treatment of hepatic primary and metastatic lesions have revolutionized the field of therapeutic nuclear medicine.
Table 14.1
Therapeutic applications of nuclear medicine
Oncologic |
1. Lymphomas and leukemias |
2. Polycythemia rubra vera |
3. Solid tumors (thyroid, ovarian, prostate and breast carcinomas, neuroblastoma, osteogenic sarcoma, and others) |
4. Treatment of metastasis-induced bone pain |
Non-oncologic |
1. Benign thyroid disease particularly hyperthyroidism |
2. Radionuclide synovectomy |
3. Bone marrow ablation |
4. Intravascular radionuclide therapy for prevention of restenosis |
It is not the objective of this chapter to discuss different protocols and experiences in the treatment of various conditions using radioisotopes. Rather, the main objective is to explore some of the pathological features of the disease processes being treated, the underlying theory behind the action of the radioisotopes that induce therapeutic effects.
14.2 Treatment of Hyperthyroidism
Since the 1940s radioactive iodine-131 (131I) therapy has been a major component of the treatment of hyperthyroidism and differentiated thyroid cancer. It has become the most common definitive treatment of hyperthyroidism and the modality of choice in treating Graves’ disease, with the result that surgeons are becoming less and less experienced in thyroidectomy since the number of operations has decreased significantly. In a recent Canadian survey study, endocrinologists were found to be the most common to prescribe 131I for malignant, while nuclear medicine physicians were the most common in prescribing it for benign disease [1].
The normal thyroid gland utilizes iodine for the synthesis of thyroid hormones (see Chap. 7). The cells of the gland do not differentiate between stable iodine and radioactive iodine. Accordingly, if radioactive iodine is administered, it is trapped and then organified by thyroid follicular cells exactly like nonradioactive iodine.
14.2.1 Pathophysiology
After oral administration, 131I-iodide is absorbed rapidly from the upper gastrointestinal tract, 90 % within 60 min. After entering the blood stream, the iodide is distributed in the extrathyroid compartment similar to the stable iodide and leaves this compartment to be taken up by mainly the thyroid and excreted predominantly by the kidneys. Approximately 20 % of the administered activity is taken up normally by the thyroid gland. A small amount of 131I is also found in the salivary glands, gastric mucosa, choroid plexus, breast milk, and placenta. Up to 75 % is excreted by the kidney and 10 % by fecal excretion. Approximately 40 % of the administered activity has an effective half-life of 0.43 days, while 60 % has an effective half-life of 7.6 days.
Graves’ disease is the most common form of hyperthyroidism, comprising approximately 56 % of all cases. It is also the major immunologically mediated form. It occurs most commonly in young women and is characterized by symptoms of hyperthyroidism with or without ophthalmopathy and dermopathy. Rarely, lymphadenopathy and splenomegaly may be present. The thyroid gland is usually diffusely enlarged but sometimes normal in size. The condition is an autoimmune process with autoantibodies directed against the TSH receptors on thyroid follicular cells which may be stimulatory and/or destructive [2]. Thyroid stimulatory antibodies include the long-acting thyroid stimulator (LATS). This antibody is detected in most patients with Graves’ disease and behaves like TSH, stimulating the production of thyroid hormones and consequently trapping and organifying radioiodine. The other stimulatory antibody is the LATS protector, the antibody that prevents degradation of LATS; accordingly, it helps to stimulate thyroid cells indirectly. The disease may be associated with other autoimmune disorders such as pernicious anemia and myasthenia gravis.
Graves’ disease is also known to be associated in Caucasians with HLA B8, DR2, and DR3 and with an inability to secrete certain glycoproteins coded for on chromosomes 6 and 19. A 50 % concordance rate is seen among monozygous twins, while 5 % concordance is noted in dizygous twins. These facts suggest a genetic susceptibility for the disease. The observation that Yersinia enterocolitica and Escherichia coli and other gram-negative organisms contain TSH-binding sites raised the possibility that the initiating event in the pathogenesis of the disease may be infectious in genetically susceptible individuals.
Histologically, there is hyperplasia of the thyroid epithelium, sometimes with papillary unfolding. Lymphocytic infiltration is present, usually less than in other forms of autoimmune diseases as postpartum thyroiditis. Little colloid storage is also seen. With time, the untreated gland will show progressive fibrosis, and the end stage will lead to hypothyroidism, which may be considered part of the natural history of the disease [3, 4].
Thyroid scintigraphy shows uniform uptake throughout the gland or, less commonly, varying degrees of nonuniform uptake. This nonuniformity is related predominantly to different stages of involution of the disease with variable amounts of fibrosis based on the duration of the disease or the presence of nodules (Fig. 14.1). The presence of a TSH-dependent functioning nodule in a diffusely toxic gland has been referred to as Marine-Lenhart syndrome (Fig. 14.1). Since the function of such nodule is much less than the surrounding hyperfunctioning tissue, it usually appears scintigraphically cold.
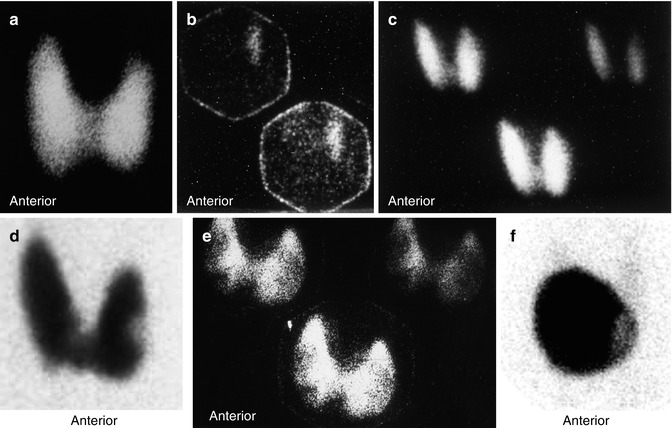
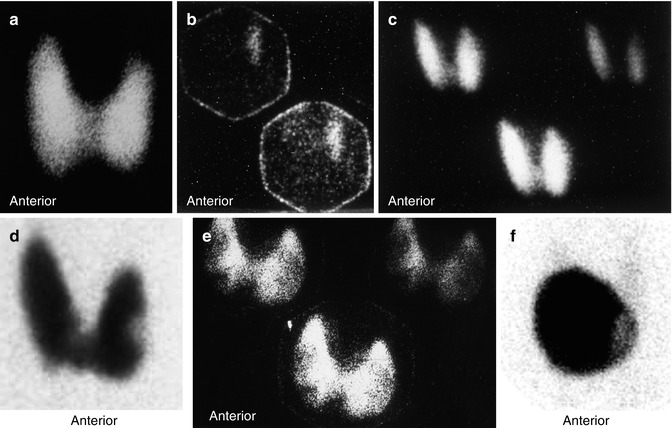
Fig. 14.1
Examples of thyroid scans of patients with hyperthyroidsim illustrating patterns that affect the treatment strategy using 131I. (a) illustrates pattern of uniform uptake in a patient with Graves’ disease. Note that scan of patients during recovery phase of thyroiditis may simulate Graves’ disease scintigraphically and show high uptake. Example (b) is of a patient with subacute thyroiditis. Initial scan (c) shows decreased and nonuniform uptake with a 24-h uptake of 1 %. Follow-up scan (b) shows uniform uptake throughout the gland with an uptake of 38 %. This may be confused for Graves’ disease if the patient is referred first during this phase. Example (d) shows a scan of a patient with Graves’ disease and a colloid nodule illustrating another pattern of “Marine-Lenhart” syndrome which is more resistant to 131I therapy. Example (e) shows diffusely toxic gland with significant nonuniformity and multiple cold nodules. Compare this pattern to that of multiple toxic nodules (Chap. 7, Fig. 7.2). This pattern also needs to increase activity per gram of tissue for successful treatment. Example (f) is for autonomous single toxic adenoma which is treated by relatively high activity
Ophthalmopathy occurs in approximately 50 % of patients with Graves’ disease [4]. Infiltration of extraocular muscles by an inflammatory reaction consisting predominantly of lymphocytes is the main pathological feature of ophthalmopathy. These lymphocytes are believed to be sensitized to antigens common to the orbital muscles and thyroid gland. Similar inflammatory infiltrates may also be present in the dermis, causing the dermopathy or pretibial myxedema which may be present in up to 10 % of patients with unclear etiology.
Single thyroid nodules can, via an autonomous function, secrete sufficient thyroid hormone to cause hyperthyroidism. These nodules are usually greater than 3 cm in diameter in order to be capable of producing this level of function [5]. Hyperfunction may also arise in a gland containing multiple nodules [6]. In this case the secretion of thyroid hormones can be either from hyperfunctioning nodules that are assumed to be autonomous or from the internodular parenchyma, which may be an expression of Graves’ disease in an otherwise nodular goiter. The nodules in the latter situation may be cold or a mixture of cold and hot, hypertrophic nodules. The term Plummer’s disease, or toxic nodular goiter, has been used to designate hyperthyroidism in glands with both single and multiple toxic nodules. The term nodular toxic goiter may be reserved for a toxic gland that contains nodules that are not hyperactive. The presence of cancer in toxic nodular goiter is extremely rare and varies from 0.1 to 0.9 %. The toxic nodular goiter may have a cold nodule representing a TSH-dependent adenoma. Scintigraphic imaging cannot exclude malignancy in the cold nodule that is not TSH dependent.
14.2.2 Mechanisms of Therapeutic Effects
The therapeutic effects of 131I-sodium iodide are due to the emission of ionizing radiation from the decaying radionuclide. In benign conditions such as Graves’ disease, division of some metabolically active cells is prevented by the effect of this ionizing radiation. Cell death is another mechanism activated when the cells are exposed to high levels of radiation, particularly when high doses are given to patients with toxic adenoma, where the suppressed normal thyroid tissue is essentially spared with delivery of a very high concentration to the cells of the toxic nodule. Cell death is followed by replacement with connective tissue, which may lead to hypothyroidism, depending on the number of cells destroyed and replaced by fibrous nonfunctioning tissue. Since 90 % of the radiation effects of 131I are due to beta radiation, which has a short range in tissue of 0.5 mm, the extrathyroid radiation, and consequently the side effects, is minimal. It has been estimated that 15 % of patients treated with 131I may show worsening of ophthalmopathy [7, 8]. Since posttreatment hypothyroidism has been associated with exacerbation of ophthalmopathy, lower-dose radioactive iodine or starting replacement hormones early (2 weeks) after therapy along with the use of prednisone 40–80 mg per day tapered over 3 months may prevent severe eye disease in up to two thirds of patients [9, 10]. It is interesting that cigarette smoking has been also implicated as a risk factor for progression of Graves’ ophthalmopathy [8].
14.2.3 Factors Affecting the Dose of 131I Used for Therapy
Several factors affect the therapeutic dose to be administered to patients suffering from hyperthyroidism. These include some parameters related to the patient, such as age, sex, medical history, and duration of treatment with antithyroid medications, and factors related to the gland itself, particularly its size, the level of radioiodine uptake, scintigraphic findings of uniform or nonuniform uptake, and whether nodules are present. Additionally, the dose is dependent on how the therapist defines the goals of therapy. If the control of thyrotoxicosis is the most important consideration, the total dose or the dose per gram of estimated thyroid tissue weight will be higher than when the therapist is trying to avoid or delay hypothyroidism [11]. Using empirical low-dose iodine therapy to avoid hypothyroidism has been shown to result in persisting hyperthyroidism in up to 54 % of patients [12]. Additionally it has been found that the rate of hypothyroidism is not different among those treated with low-dose and high-dose radioiodine [13, 14].
14.3 Treatment of Differentiated Thyroid Cancer
Radioactive iodine is the mainstay of therapy for residual, recurrent, and metastatic thyroid cancer that takes up iodine and cannot be resected [13]. The tissue of normal thyroid and its tumors expresses a variety of oncogenes, growth factors, and growth factor receptors. There is increased expression of some oncogenes, namely, c-myc/c-fos and c-ras, in some epithelial and medullary thyroid carcinomas.
C-myc mRNA and c-fos mRNA are found in high levels in papillary carcinomas compared with the surrounding normal thyroid tissue. Patients with an unfavorable prognosis were twice as likely to overexpress c-myc as patients with good prognosis [15].
Ras oncogenes were found in 80 % of follicular and 20 % of papillary carcinomas. This high prevalence of transforming ras oncogenes in follicular carcinomas may explain its aggressive behavior in comparison to papillary carcinoma and may suggest a role of this oncogene in the metastatic phenotype of this cancer [16]. Recently a tissue-specific oncogene associated with papillary carcinoma has been identified.
Excessive growth factor and increased expression of oncogenes encoding growth factors or growth factor expression, such as the oncogene of c-ras B, were identified in papillary carcinoma, adenomas, and anaplastic carcinoma.
Besides the importance of growth factors in the development of thyroid carcinoma, links have also been found to certain risk factors. The most important of these is radiation exposure. Exposure to radiation following the explosion of the atomic bombs in Japan, as well as after head and neck radiation, resulted in a 30-fold increase in the incidence of thyroid cancer [17].
About 90 % or more of thyroid carcinomas are well differentiated, of the papillary, papillofollicular, follicular, and Hürthle cell types, which take up iodine and accordingly can be successfully treated with 131I. The therapeutic effects on differentiated thyroid cancer, where larger doses of radioactive iodide are administered, are based on destruction of cells of the residual thyroid tissue and the functioning carcinoma cells by the high dose of administered radionuclide. The mortality of patients treated with less than total thyroidectomy and limited 131I therapy was found to be three to four times higher than that of patients treated with total thyroidectomy and 131I therapy (Fig. 14.2) to ablate known foci of radioiodine uptake [18]. Because of the larger dose of radionuclide and the lower uptake by the tissue in the case of thyroid cancer, more side effects can be seen, particularly transient sialadenitis, than in treatment of hyperthyroidism. This however does not justify using limited therapy such as 30 mCi. A recent study confirmed the high rate of efficiency of the high ablative dose of 100 mCi of 131I particularly in patients with less than 2 % neck uptake values [19]. This study confirmed also that success rate is dependent on the pre-therapy neck uptake. The success rate was 94 % when pre-ablation uptake was less than 2 %, 80 % with uptake between 2 % and 5 %, and 60 % when uptake value was more than 5 % [19].
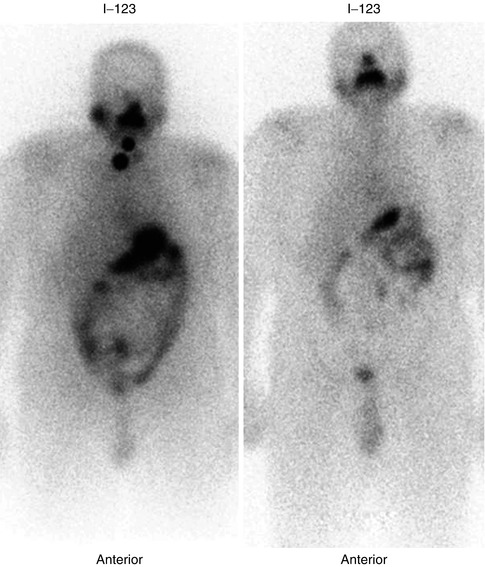
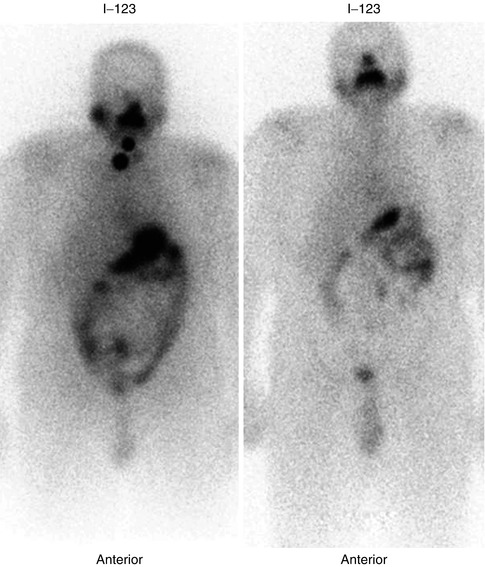
Fig. 14.2
Follow-up 123I whole-body scan in a patient with papillary thyroid carcinoma treated with total thyroidectomy and 131I ablation showing resolution of the neck activity 1 year after 131I postoperative ablation
Thyroglobulin and calcitonin are the major tumor markers for thyroid cancer of the follicular epithelium and parafollicular C cells, respectively. These markers are unique, in the sense that they are not only specific for tumor tissue but are also specific components of normal thyroid tissue. Thyroglobulin is an iodinated glycoprotein essential for synthesis and storage of thyroid hormones. Since thyroglobulin is produced exclusively by thyroid tissue, only very small amounts can be found in the blood after thyroidectomy and ablative radioiodine therapy. Accordingly, any post-therapeutic elevation of its levels indicates either remnant thyroid tissue, requiring further ablative treatment, or the presence of metastases or local recurrence. Other tumor markers used for many other tumors, such as carcinoembryonic antigen (CEA) and tissue polypeptide antigen (TPA), are not specific for thyroid cancer. TPA, which is a cytokeratin-related nonspecific proliferation marker, has a sensitivity of 40–60 % for thyroid cancer. However, it has a good correlation with tumor progression or therapeutic response, with a high positive predictive value of 90 %. Evaluation of ablative therapy and follow-up of patients post-ablation to monitor disease recurrence has been further improved and is facilitated by the availability of recombinant human thyrotropin as well as the use of 18 F-FDG positron emission tomography. The value of recombinant human thyrotropin (rhTSH) rests on providing the opportunity to obtain diagnostic whole-body 131I scan under adequate TSH elevation as well as representative thyroglobulin levels while the patients are receiving their thyroid hormone [20]. FDG PET is useful in evaluating patients in instances where radioiodine imaging fails to identify known or suspected recurrent or metastatic disease [21]. Additionally the use of 201Tl and 99mTc-MIBI particularly when FDG PET is not available is of value for this purpose [22].
14.4 Treatment of Pain Secondary to Skeletal Metastases
Approximately 75 % of patients with advanced cancer have pain, with a high percentage due to skeletal metastases. Bone metastases cause intractable pain, which affects the quality of life for the patient, especially if it is associated with immobility, anorexia, and anxiety, with the consequent long-term use of narcotic analgesics. The mechanism of bone pain may not be clear in many of these patients and could be due to cell-secreted pain modulators such as interleukin-1 beta, interleukin-8, and interferon [23]. Depending on the extent of bone metastases, radiation therapy or radiopharmaceuticals can be used instead of narcotics to alleviate the pain with the objective of improving the quality of life.
Radiotherapy for focal painful metastases with delivery of 2,000–3,000 rads induces pain relief in 60–90 % of cases [24, 25]. Controlling pain of multiple metastases using external beam radiotherapy is difficult. Hemibody irradiation using 800 rads to the lower half of the body and 600 rads to the upper half has resulted in complete response in 30 %, partial response in 50 %, and no response in 20 % of patients. Radiotherapy used for painful skeletal metastases often produces significant side effects such as nausea, vomiting, and diarrhea, as well as bone marrow toxicity in one third of patients. Vomiting and diarrhea can be severe in 10 % of cases, and hematological side effects can be life threatening in approximately 9 % of patients [26].
Bone-seeking radiopharmaceuticals emitting beta particles have been used to deliver local radiotherapy to metastases to decrease pain at their sites. Radiopharmaceuticals which are taken up at the sites of bone metastases will cause less toxicity than external radiation therapy. These radiopharmaceuticals control pain while causing only transient bone marrow depression, which is usually mild. The uptake of these radiopharmaceuticals by metastases is severalfold (up to 15–20 times) that of normal bone. These agents are absorbed to hydroxyapatite crystals at the site of active new bone, similar to 99mTc-MDP. They include phosphorus-32, strontium-89, rhenium-186 diphosphonate, and samarium-153 EDTMP. The list of radiopharmaceuticals for bone palliation has been increasing including 188Re, 177Lu, and others [27]. Clinical trials using 223Radium and/or combinations of chemotherapy and radionuclides are aiming at a more curative approach [28].
14.4.1 Radiopharmaceuticals
Sr-89 chloride is a pure beta-emitter with a relatively long half-life of 50.5 days. It is a chemical analogue of calcium, and accordingly it concentrates avidly in areas of high osteoblastic activity. Samarium-153 is produced in the nuclear reactor by neutron activation of both natural Sm-2O3 and 98 % enriched Sm-152 targets. It has a relatively short half-life of about 48 h. Coupling of the radionuclide to ethylene diamine tetramethylene phosphonate (EDTMP) leads to the high uptake of the radionuclide by bone. Gamma camera imaging is possible due to the 103 keV gamma ray emitted during decay of Sm-153.
Samarium-153 is produced in the nuclear reactor by neutron activation of both natural Sm-2O3 and 98 % enriched Sm-152 targets. It has a relatively short half-life of about 48 h. Coupling of the radionuclide to ethylene diamine tetramethylene phosphonate (EDTMP) leads to the high uptake of the radionuclide by bone. Gamma camera imaging is possible due to the 103 keV gamma ray emitted during decay of Sm-153.
Phosphorus 32 orthophosphate is used uncommonly for the treatment of bone metastases. Dosimetric studies have demonstrated a relatively high dose to the bone marrow from the highly energetic beta particles of this radionuclide causing myelosuppression with pancytopenia.
Tin-117 m is a reactor produced radionuclide, with a half-life of 13.6 days. Tin-117m is linked to diethylenetriaminepentaacetic acid (DTPA). More than 50 % of the administered activity is absorbed by bone in patients with metastatic carcinoma with a bone to red marrow ratio of up to 9:1. Contrary to the other radionuclides mentioned above, this radionuclide emits internal conversion electrons. Its 159 keV photon energy allows correlative imaging with a similar uptake pattern as Tc99m-MDP.
14.4.2 Mechanism of Action
Metastatic bone pain is believed to be due to mechanical factors due to local bony destruction and to humoral factors resulting from secretion of certain mediators by tumor and peri-tumoral cells (Table 14.2). Although the mechanism of action of these radiopharmaceuticals in relieving bone pain is not completely known, the therapeutic effect is thought to be achieved by delivering sufficient energy from the sites of reactive bone directly to the cells of metastases and/or to peri-tumor cytokine-secreting cells that may be responsible for the patient’s pain. Pain relief by radiation was found to be independent of the radiosensitivity of the tumor, and, therefore, the mechanism of action does not involve actual killing of the tumor cell. It is more likely that radiation interrupts processes that are maintained by humoral pain mediators in the microenvironment of the tumor [29]. This view is also supported by absence of a dose-response relationship [30].
Table 14.2
Possible mechanisms of metastases-induced bone pain
1. Stretching periosteum |
2. Pressure on nerves |
3. Direct bone invasion and local destruction |
4. Cell-secreted pain modulators such as interleukin-1 beta, interleukin-8, and interferon |
14.4.3 Choice of Radiopharmaceutical
It has been demonstrated that myelosuppression is less severe using radionuclides with relatively shorter half-lives favoring the use of 153Sm, 186Re, 117Sn, and 89Sr. Other physical properties including radiolabeled conjugate biological uptake and clearance, product-specific activity, range and type of emissions, and resultant effects on tumor and normal tissue cellular survival should be all considered along with the clinical outcome to choose a radiopharmaceutical. The response rate of different radiopharmaceuticals currently in use appears not to differ significantly [31]. The side effects which are mainly hematological vary among the agents used, being more pronounced with 32P than with the newer agents. Tin-117m DTPA differs from the other radiopharmaceuticals in that its emission is internal conversion electrons rather than beta particles. Since internal conversion electrons have low energy and a shorter path in tissue, they may result in less marrow toxicity.
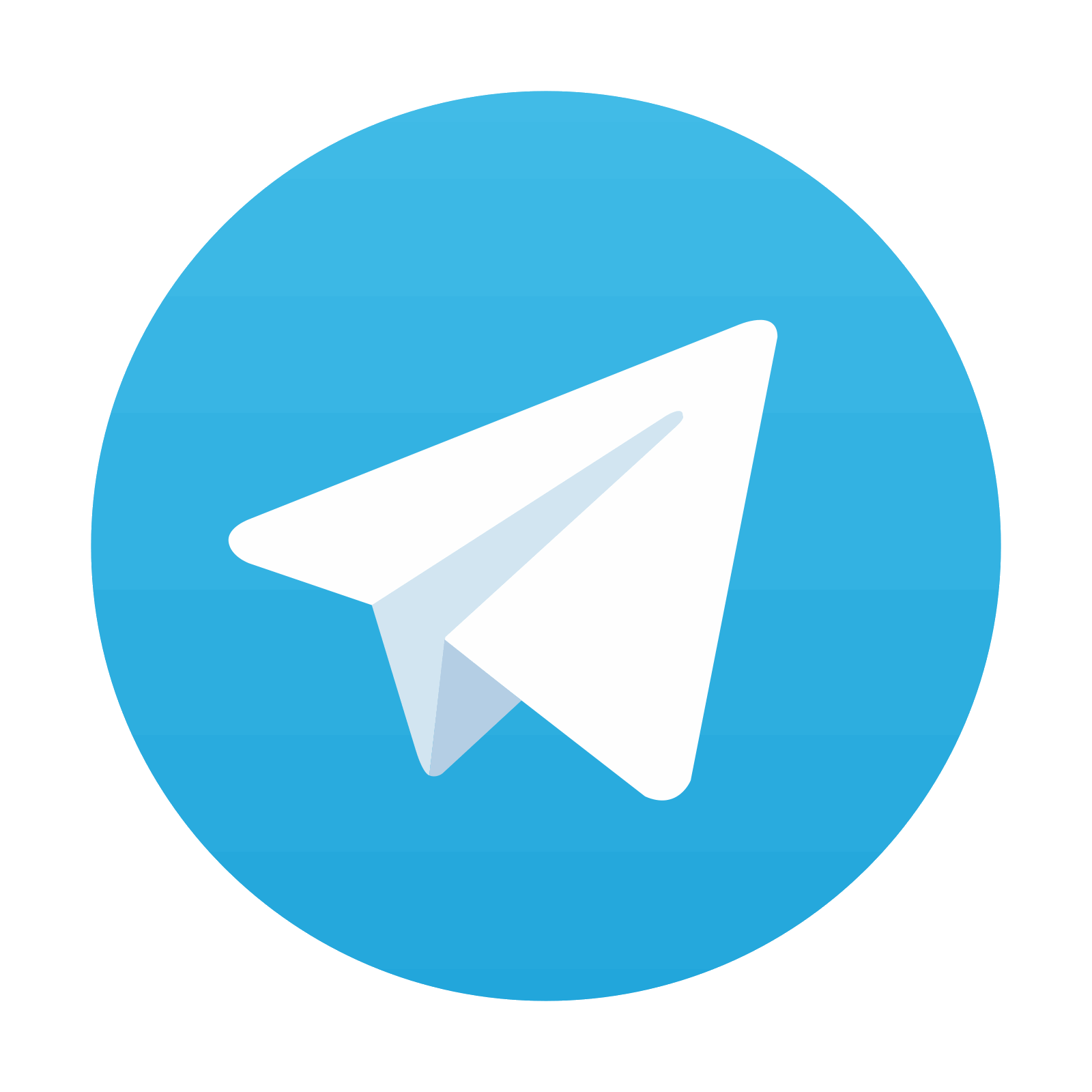
Stay updated, free articles. Join our Telegram channel
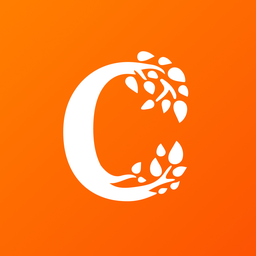
Full access? Get Clinical Tree
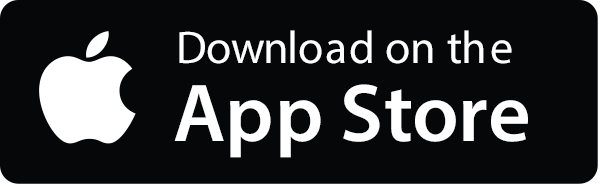
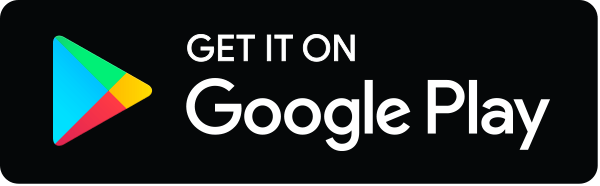