Fig. 1.1
The illustration shows the acquired biologic characteristics of malignant tissues proposed by Hanahan and Weinberg that has helped to understand the fingerprints of cancer cells (From Hanahan and Weinberg [3] with permission)
1.1.1.1 Sustaining Proliferative Signaling
One of the fundamental attributes of cancer cells is their capability to proliferate even with no associated signaling. This is what Hanahan and Weinberg called sustaining proliferative signaling and can be achieved by increasing the production of growth factors, stimulating normal cells to provide cancer ones with growth factors, activating protein in the downstream signaling pathway, and increasing the number of receptors on the cell or either modifying them to ease cancer cell signaling. In cancer cells, the process of intracellular communication may be taken over by specific mutations in otherwise normal genes, resulting in an abnormal gene called oncogene. Oncogenes generate abnormal genes products such as oncoproteins leading to a malignant cell behavior. These mutations may be produced in kinases, including EGFR mutations and c-Kit mutations, among others, that can disturb the signal transmitting function [1]. PI3K-Akt signaling, MAP-kinase pathway, mTOR pathway, cellular senescence, or oncogenes and tumor suppressor genes might contribute in this step [3]. This is key in targeted drug therapy as there is an interaction of membrane receptor-based tyrosine kinase and PI3K-Akt and RAS/ERK pathways and many drugs being used to inhibit signaling in different ways. Molecular-based imaging modalities have been used to image these molecular pathways. For example, some studies have demonstrated the extraordinary effect against gastrointestinal stromal tumors (GIST) of a tyrosine kinase inhibitor imatinib drug (Gleevec). GIST has been related to a transmembrane receptor, the oncogenic protein c-Kit. 18F-fluorodeoxyglucose or 18F-FDG, an analogue of glucose labeled with a positron emitter F-18, has been used in molecular imaging for assessing the therapeutic response of this drug in GIST patients. 18F-FDG has shown the effect of blocking the specific tyrosine kinase activity associated with c-Kit [1]. Depending on the drug being used and its molecular target, a certain type of radiopharmaceutical can be applied to assess treatment response. For example, other tyrosine kinase inhibitor drugs, sunitinib and sorafenib, have been used in a type of kidney cancer. These drugs are targeting a specific angiogenic growth factor called vascular endothelial growth factor or VEGF. Other tracers such as 18F-desatibib, 68Ga-Fab’2 herceptin, and 18F-fluorodihydrotestosterone or 18F-FDHT have been used to image different targets: mutant ABL in resistant chronic myeloid leukemia (CML), Her2 in breast cancer, and androgen receptor (AR) in prostate cancer, respectively.
1.1.1.2 Evading Growth Suppressors
Cell proliferation in normal cells is a controlled process where many signals (pro- and anti-growth) coordinate the cycle phases. For example, the G1 phase is the most vulnerable cell cycle vital due to the fact that it is at this point where extrinsic mitogenic signals may facilitate more mutations and therefore enable the development of cancer cells [4]. The rapid growth of malignant tissue can be measured by computed tomography imaging (CT) to evaluate changes in volume. Molecular imaging with specific tracers linked to proliferative processes such as the accelerated synthesis of DNA is more suited for this. Many tracers have been tested to image this mechanism. 2-18F-fluorothymidine or 18F-FLT is probably the most interesting and extensively used radiopharmaceutical in this setting. 18F-FLT is a pyrimidine-based tracer taken by the cell via passive diffusion and facilitated transport by Na+-dependent carriers and then phosphorylated by thymidine kinase 1 (TK1) and finally trapped in the cell. In quiescent cells, TK1 activity is virtually absent, but in proliferating cells its activity is increased, particularly in the S phase of the cell cycle. This radiolabeled tracer has shown very promising results in monitoring response to treatment. In one study performed by Pio et al., 14 breast cancer patients were evaluated 2 weeks after the first cycle of treatment. Levels of the cancer antigen 27.29 (CA27.29), which is a soluble form of a mucin-like glycoprotein abundantly expressed on most carcinoma cells, showed very good correlation with 18F-FLT uptake, as shown in Fig. 1.2 [5]. Another study evaluated 18F-FLT in early response evaluation of high-grade non-Hodgkin lymphoma in 22 patients that were treated with combined immunochemotherapy (R-CHOP) or chemotherapy (CHOP) alone showing that a rapid reduction in FLT uptake was shown 7 days after initiation of therapy. However, there was no significant change in FLT uptake following the administration of rituximab alone [6].
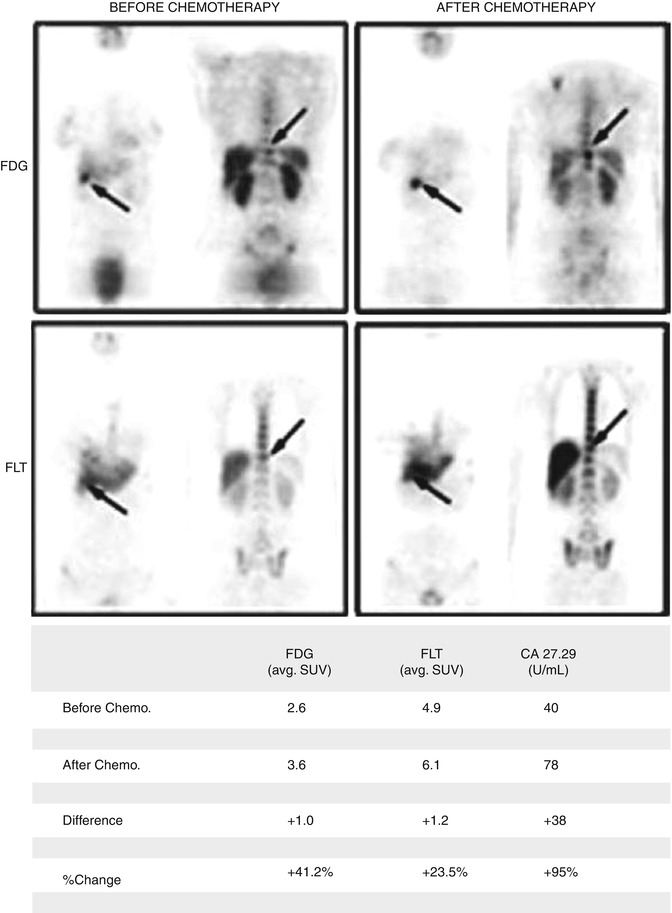
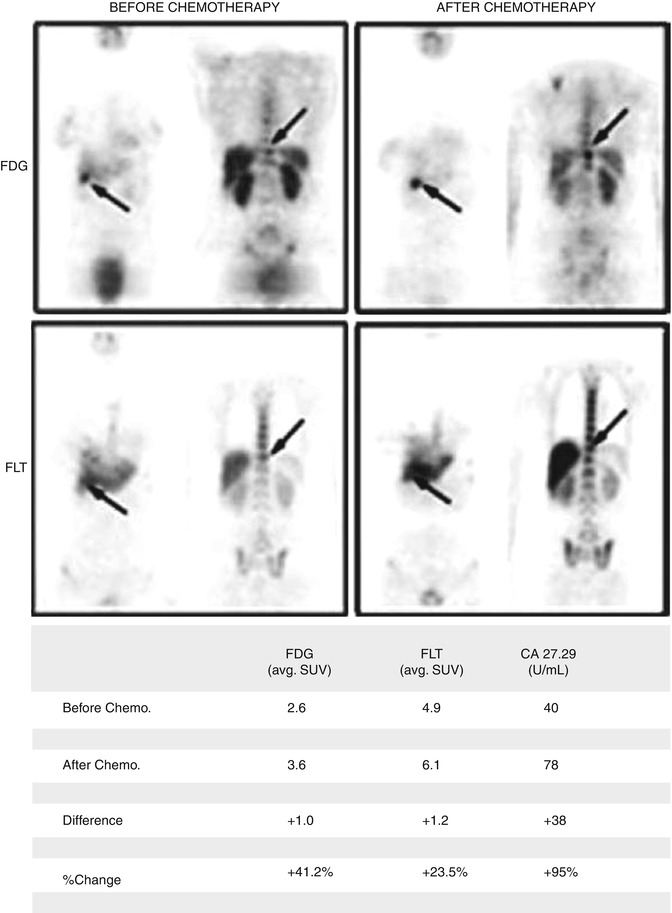
Fig. 1.2
PET studies of a 43-year-old female with known metastatic breast carcinoma. 18F-FDG-PET and 18F-FLT-PET were performed before and after Aromasin treatment (an aromatase inhibitor). Both FDG and FLT studies show an increase of radiotracer uptake in the right kidney between the pre- and posttreatment scans. In addition, levels of tumoral biomarker CA27.29 also increased (From Pio et al. [5] with permission)
The way antiproliferative signals usually work in normal cells is by inducing the G0 phase or a postmitotic state [4]. However, most cancer cells evade these signals so they can proliferate. This characteristic is known as evading growth suppressors [3].
The two most representative tumor suppressors are p53 and the retinoblastoma protein (Rb) which regulate the cell cycle. While the protein p53 operates as a central control of apoptosis when there is a DNA damage, the Rb protein decides whether a cell should go or not through its cell cycle; in other words, it inhibits the pathway through the restriction checkpoint in G1 [3, 7]. A protein such as p53 has been thought to participate in cancer cells metabolism as a modulator, especially by facilitating oxidative phosphorylation and reducing glycolysis. Therefore, when p53 is inactive due to a mutation, a process of glycolysis is then favored. This process can be studied with 18F-FDG as this is a marker of glucose metabolism as some authors have shown a correlation between p53 and 18F-FDG [8–10]. When any of the tumor suppressors or processes is dysregulated, then the cycle progresses and consequently the cell proliferation is ongoing.
1.1.1.3 Activating Invasion and Metastasis
Another important cancer hallmark is activating invasion and metastasis, responsible for the spreading of neoplastic cells from the primary site into surrounding tissues and distant organs. It is believed, though not clear, that this process involves modifications in the cells so they can be attached to other cells and to the extracellular matrix (ECM) [3]. This may include different stages: local tissue invasion, intravasation, transition via blood and lymphatics, and colonization of foreign tissue [3]. Matrix metalloproteinases (MMPs) consist of a family if zinc-dependent endopeptidases for degrading ECM constituents, playing an important role in tumor invasion and metastases. The overproduction and uncontrolled function of MMPs have been correlated to many different tumors (brain, colon, melanoma, breast, etc.) [11]. Cross talk between cancer cells and cells of neoplastic stroma introduces the idea that metastatic process requires input from the surrounding tissue, so they do not originate from a cell-autonomous model [3, 12]. Imaging MMP expression with noninvasive imaging techniques such as PET, single-photon emission tomography (SPECT), and optical imaging may offer important information to predict metastatic potential of a tumor. Some synthetic sulfonamide-based MMP inhibitors including 64Cu-DOTA-CTT, 64Cu-DOTA-STT, and 123I-CGS 27023A, among others, have been used as radiotracers to image overexpression of MMPs in tumors [11, 13].
1.1.1.4 Inducing Angiogenesis
The formation of new blood vessels, also called angiogenesis, is an essential process of tumor growth and metastatic dissemination [14]. Generally, angiogenesis is strongly controlled by a balance between stimulatory and inhibitory signaling molecules. However, when this balance is disrupted, a rapid proliferation of new vessels can happen, for instance, when a primary tumor releases angiogenic growth factors. The tumor-associated neovasculature is a multistep mechanism including signaling input from multiple angiogenic growth factors like VEGF, known as “angiogenic switch,” and also the integrin signaling pathway [3]. Integrins are a group of cell adhesion molecules that are present on both neoplastic cells and newly formed vessels. Among the different integrin molecules already known, αvβ3 is the most studied in angiogenesis. Angiogenesis is therefore essential to the survival and growth of neoplastic tumors. Inducing angiogenesis eases tumor expansion mainly by delivering of oxygen and nutrients and production of growth factors for the cancer cell [14]. Angiogenesis has attracted some attention in therapy as a target for chemotherapeutic drugs. In fact, the first anti-angiogenic drug proven in humans was bevacizumab, a humanized monoclonal antibody which targets VEGF [15].
These processes can be imaged with radiolabeled tracers such as 64Cu(DOTA)-VEGF121 (targeting VEGF) or 18F-galato-RGD (targeting integrin αvβ3) for PET imaging [16, 17]; 125I-VEGF and 111In-perfluorocarbon for SPECT imaging [18]; NP-RGD for SPECT imaging; RDG-Gd3+ for magnetic resonance imaging (MRI); RGD MBs and anti-VEGFR-2-Ab-microbubbles for ultrasound (US) or RGD-QD705; and QD-VEGF(DEE) for optical imaging (OI) [19].
1.1.1.5 Perfusion
The perfusion of tumors and their surrounding tissues is considered an essential physiological characteristic of tumor microenvironment as it is meant to be very important for planning and monitoring treatment response. Many imaging modalities including CT, MRI, and radionuclide techniques have shown their utility in understanding perfusion not only in oncology but also in heart pathologies (myocardium viability) and neurodegenerative disorders (dementia). Several tracers have been used to study blood flow, hypoxia, and neovascularization in primary tumors to investigate tumor perfusion. For example, 15O-H2O has turned out to be one of the most promising tracers to explain tumor perfusion in breast, kidney, colon, or lung cancer [20–22]; 62CuIIPTSM or other more commonly used PET perfusion imaging agents such as 82Rb-RbCl, 13N-NH3, and 64Ga-citrate have shown potential in brain, myocardium, and kidney, although with limitations [1]. Different CT techniques and novel MRI sequences are being used to learn more and quantify perfusion in neoplastic tissues and will be detailed in Sect. 1.2.
1.1.1.6 Resisting Cell Death
The programmed cell death (apoptosis) is a key process in cancer development and progression. In normal cells when an event such as an irreparable DNA damage is produced, they tend to switch on apoptosis. The ability of cancer cells to avoid apoptosis and continue with their proliferation is one of the fundamental features of cancer and is a major target of cancer therapy development [2, 3]. A signaling dysregulation in cancer cells promotes the overexpression of antiapoptotic proteins and mutes the production of proapoptotic proteins [23]. Moreover, by altering the necrosis and normal cellular autophagy, cancer cells may resist cell death [3]. A radiolabeled molecule to image apoptosis called 18F-ML10 has shown promising results in brain metastasis from non-small cell lung cancer patients treated with radiation therapy with promising results [24].
1.1.1.7 Hypoxia
Related to apoptosis, angiogenesis, tumor invasion, and metastasis, hypoxia is another very interesting feature that has impact on tumor environment. Hypoxia is a pathological condition defined as a reduction of tissue oxygenation and may lead to an insufficient supply of oxygen and nutrients to cells. In oncology, its severity will be dependent on the phenotype of cancer. For instance, cervical cancers are known to be highly hypoxic [1]. It has been exhibited in many studies that hypoxic neoplasias have worse prognosis for disease-free survival after treatment with chemotherapy as cytotoxicity aimed to be produced with certain anticancer drugs decreases in hypoxic tissues [25, 26]. Furthermore, hypoxic cells show significantly more resistance to radiotherapy treatment so affecting the radiation sensitivity [27–30]. Several hypoxia-selective PET tracers have been used with different results, but it is worth highlighting 18F-fluoromisonidazole (18F-MISO) and 64CuII-ATSM which are being tested in many studies with interesting outcomes [31, 32]. 18F-MISO has affinity for hypoxic cells with functional nitroreductase enzymes; therefore, it accumulates in activated cells but not in necrotic cells [33]. Many studies have shown excellent correlation between 18F-MISO uptake and the oxygenation status of non-small cell lung neoplasms, head and neck cancer, gliomas, and cervical cancer [34].
Another interesting radiopharmaceutical is 124I-cG250, an iodine-based radiolabeled tracer, which has shown the potential in evaluating hypoxia in certain tumors. cG250 is an antibody reacting against carbonic anhydrase-IX, which is overexpressed in hypoxic conditions, especially in clear cell renal carcinomas, the most common and aggressive renal tumor [35, 36]. Divgi et al. studied 26 patients with renal masses who were scheduled to undergo surgical resection. They concluded that PET with 124I-cG250 could identify accurately clear cell renal carcinoma; therefore, a negative PET scan would be highly predictive of a less aggressive phenotype helping surgical planning (Fig. 1.3) [37].
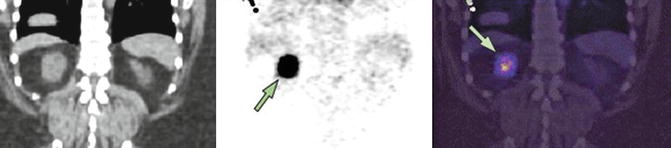
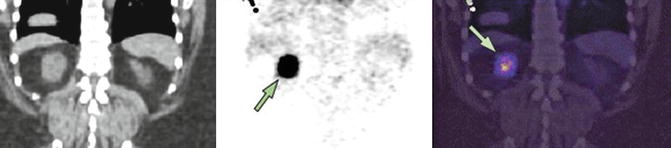
Fig. 1.3
Patient with a clear cell carcinoma scanned with 124I-cG250 PET/CT (coronal CT, PET, and fused image) showing a focus of abnormal uptake (arrow) in the posterior portion of the right kidney (From Divgi et al. [37] with permission)
1.1.1.8 Deregulating Cellular Energetics and Avoiding Immune Destruction
In addition to all those oncologic features, two new hallmarks have been recently introduced in oncology: deregulating cellular energetics and avoiding immune destruction, together with some enabling characteristics such as the genome instability and mutation generating random mutations and the tumor-promoting inflammation that favors tumor progression through various ways (Fig. 1.4) [3].
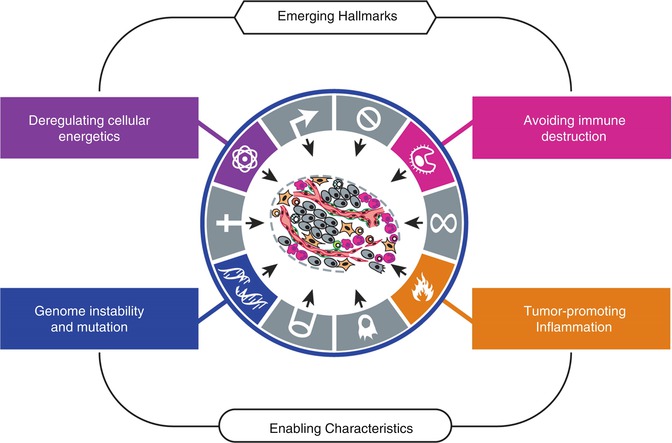
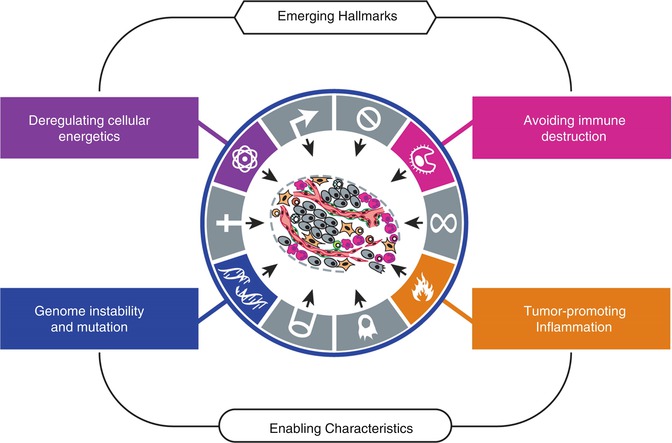
Fig. 1.4
The figure presents new proposed additional hallmarks that are thought to be involved in the pathogenesis of most cancers: one could have the ability to modify cellular metabolism by reprogramming or modifying it, while the other hallmark would be based on the capability of avoiding immunological destruction by cancer cells. In addition, the authors introduced two characteristics of neoplastic cells that could facilitate the acquisition of core and those emerging features: genomic instability and mutation, and inflammation, both of them enabling tumor progression (From Hanahan and Weinberg [3] with permission)
Cancer cells must make adjustments in their energy production in order to maintain the uncontrolled proliferation. Many mechanisms including using alternative metabolic pathways, adapting their glucose metabolism, and upregulating glucose transporters (GLUT) translate into an increase glucose uptake and utilization shown in many neoplasms, easily corroborated by molecular imaging, particularly by 18F-FDG-PET [3, 42]. 18F-FDG, as an analogue of glucose, is transported into cells by glucose transporter proteins and then phosphorylated by hexokinase to form FDG-6-phospate. However, it cannot be degraded via the glycolysis pathway nor dephosphorylated by glucose-6-phosphatase like glucose is. Therefore, FDG-6-phosphate gets trapped within the cell, and in this sense the more FDG there is in the cells, the greater the uptake in the tumor.
Many studies have suggested that a continuously active immune system recognizes and eliminates the majority of cancer cells before settling to form a tumor mass [3, 43, 44]. So the immune system is considered a fundamental process preventing tumor formation.
However, this is an unresolved issue because the role of the immune system is not yet clear. Hanahan and Weinberg and other authors introduced the concept of an elimination phase where the immune system recognizes and eliminates cancer cells. Then, the immune system is able to control the cancer cell growth but is not able to eliminate other cancer cells being in an equilibrium phase. Finally, tumor cells or clones not detected or destroyed by the immune system keep on growing [3, 45].
Each of the alterations that are produced in cancer cells of these hallmarks and tumor microenvironment characteristics are associated with fundamental key molecules that might be targeted with certain probes or tracers and imaged.
1.1.2 Biomarkers
By definition, a biomarker is a biological molecule used as an imprint of a biological condition. It is a feature used to measure and evaluate, in an objective way, biological or pathogenic processes. Also it can be used as a treatment response marker, to detect or isolate a particular cell type or even a genetic level.
Biomarkers can be classified accordingly to the information gathered [46]:
(a)
Histological (or biochemical) parameters obtained from biopsy or surgery tissue samples
(b)
Biochemical parameters from blood or urine samples
(c)
Anatomical, functional, or molecular parameters obtained with different imaging modalities
Therefore, biomarkers can be of tremendous value, being an essential piece of predictive and preventive medicine mainly in cardiology, neurology, and oncology, not only by adding understanding to these pathological processes but also by offering personalized therapy targeting cancer hallmarks [3].
Depending on what we want to image and/or treat, we have to acknowledge the target. Based on the hallmarks of cancer previously commented, many biomarkers have been studied including VEGF or integrin vectors for evaluating angiogenesis, radiolabeled glucose for the abnormal cellular energetics, thymidine analogues for proliferative signaling, receptor- [47] or enzyme-based [48] imaging, antibodies, selective anti-inflammatory drugs, telomerase inhibitors for the replicative immortality of cancer cells, and apoptotic markers, among endless others.
All of the many signaling targets have been made possible thanks to a numerous researchers by implementing translational studies from laboratory, preclinical, clinical, or population cohorts into clinical applications after an extensive testing in experimental models [49].
Imaging biomarkers, or molecular imaging, have many advantages including being considered a noninvasive method; it can be quantified [50, 51], is reliable, and offers reproducible data allowing multiparametric imaging. It represents a group of methods with specific probes, contrast agents, or MR pulse sequences [49] that image particular targets or pathways. All this infinitive information can be very useful to clinicians in the daily practice.
Besides cancer-related biomarkers, one of the fields where molecular imaging has an interesting impact is in cardiac studies. Vulnerable atherosclerotic plaque can be studied with coronary angiography by looking at the coronary lumen [52] requiring catheterization or at the coronary arterial wall with intravascular ultrasound (IVUS) [53, 54], though in these cases, it is considered to be an invasive imaging method. Other intravascular imaging techniques such as coronary computed tomography and cardiac magnetic resonance have been used for coronary and carotid lumen and wall [55] imaging. Optical coherence tomography (OI) [56] and near-infrared spectroscopy used for tissue spectral contrast [57] are being investigated.
In neurology, MRI and CT have been used to study brain infarction size and extent, lesion size, brain activity in multiple sclerosis and structural atrophy, and β-amyloid plaques with PET [58–60].
Section 1.2 of this chapter will briefly go through some of the molecular imaging techniques and its biomarkers and the unthinkable possibilities that imaging may offer in cancer biology.
1.2 Imaging Modalities: Molecular Imaging
Molecular imaging is a relatively “new” area that integrates molecular biology and state-of-the-art medical imaging, arisen in the last 15–20 years as a nexus between molecular biology and in vivo imaging. As already stated, it is defined as a noninvasive technique able to visualize the natural microenvironment in real time, measure pharmacokinetics and pharmacodynamics of pharmaceuticals, and measure physiological or pathological inside living subjects at a cellular, molecular, or genetic level, among others [61–64].
Compared with conventional imaging techniques, molecular imaging has many advantages including 3D images of living organism thus enabling research into physiological or pathological situations such as signal transduction pathways, cell metabolism, and gene expression.
MI is a multidisciplinary branch of knowledge including molecular biology, genetics, biochemistry, physiology, physics, mathematics, pharmacology, immunology, and medicine [64]. Five imaging modalities are available for molecular imaging: CT, MRI, optical imaging, US, and radionuclide imaging which includes PET and SPECT.
Challenging problems remain to be solved such as the developing of new probes, translational research to the clinical arena, and multidisciplinary approach.
Each of the different imaging techniques has their different strengths and weaknesses that will be explained in the following section.
1.2.1 Computed Tomography
Computed tomography (CT) is based on the different attenuation levels in the tissues in the body produced by X-rays, thus resulting in an anatomic image. CT imaging has undergone an interesting evolution in the last few years. The development of high-resolution CT scans allows going deeper into a molecular level, a very important role in research studies [65, 66].
The main advantage of CT is probably its high spatial resolution (0.05 ~ 0.2 mm in preclinical studies and 0.5 ~ 1.0 mm in clinical studies). Availability, cost-effectiveness, fast acquisition time, excellent hard tissue imaging (bone), and relative simplicity are some of the advantages of CT imaging. On the other hand, some of the key limitations are exposure to ionizing radiation, limited soft tissue resolution, limited molecular imaging applications, and relatively low sensitivity (~10−3 mol/L). Recently, major improvements have been made to obtain significant dose reduction.
Due to the limited soft tissue resolution, CT contrasts have been developed for a better discrimination between normal and abnormal tissues. These are iodine-based contrast agents and are typically nonspecific [64, 67]. Also these contrast agents produce some renal toxicity so it cannot be used in all patients. For these reasons, a need for developing new contrast agents is being studied by many researches such as the use of targeted gold nanoparticles alone or even combined with 2-deoxy-D-glucose for a high-resolution anatomic and metabolic information of the subject scanned [68–72].
Some of the most significant and latest technological advances in CT are multislice CT, dual-energy CT, CT spectroscopy, photon counting imaging, and phase-contrast CT imaging, among others.
1.2.1.1 Multislice CT
Multislice CT came into the game with helical scanning and multi-detector CT (MDCT). The latter one can acquire an important number (recently up to 320-detector row MDCT) of tomographic slides in a very fast time [73, 74].
MDCT has the advantage of obtaining an enhanced spatial temporal resolution. Also the very short time that it takes to scan an individual avoids most of the motion artifacts produced by the patient. For this reason, it has become an important imaging tool for cardiac imaging [64].
1.2.1.2 Dual-Energy CT
Dual-energy CT (DECT) has shown a number of advantages compared to single-energy CT with energy-integrating detectors and is considered another advance in imaging. It consists of two separate X-ray tubes that can be operated at the same energy or at two different energies producing both the material decomposition images and monochromatic spectral images at energy levels ranging from 40 to 140 keV [77]. The possibility of acquiring on the distinctive K-edge energies at the same time offers tissues to be characterized not by their attenuation values but by their composition. DECT or spectral CT is able to discriminate according to the difference in attenuation between two energies. K-edge imaging allows selective and quantitative imaging of administered contrast agents equipped with appropriate K-edge materials [69]. These features enable spectral CT a high spatiotemporal resolution and quantitative molecular imaging technology.
Photon counting detector splits the X-ray spectrum into several energy bins, and separate CT data are acquired in each energy bin. The CT data acquired in different energy bins are used for material decomposition, for example, calcium, uric acid renal stones [78], evaluating malignancies [79], differentiating hypervascular hepatic lesions [80], or even checking bone mineral density [81].
Photon counting may represent the most advanced form of spectral CT. Instead of separating the beam into merely high- and low-energy ranges, photon counting uses tight number of subranges or bins of the spectrum in order to form images based on the analysis of the spectral signature of tissues.
1.2.2 Magnetic Resonance Imaging
Magnetic resonance imaging (MRI) is a comprehensive molecular imaging without ionizing radiation, offering high temporal and spatial resolution and superb soft tissue contrast. By using a combination of powerful magnet and radiofrequencies, it displays anatomical and physiological information.
Other advantages of MRI include limitless depth of penetration, quantification data, and an ample clinical utility. An important disadvantage of MRI is its low sensitivity (10−3 ~ 10−5 mol/L) [61], which lower than many other molecular imaging modalities [64] that can be very limiting due to the nonsignificant differences between atoms in high- and low-energy status.
However, efforts have been made to increase this low sensitivity by either increasing the magnetic field – 3.0 T in clinical environment and up to 7, 9.4, or even 11 T in the research field [82–84] – or hyperpolarizing atoms through dynamic nuclear polarization or by using the optical pumping technique enhancing significantly MR signals from different atoms [85, 86].
The contrast between different tissues seen on MRI is created by different relaxation times of each tissue. In order to achieve molecular imaging of disease biomarkers using MRI, targeted MRI contrast agents with high specificity and sensitivity (relaxivity) are required.
Beyond the most commonly used MRI sequences, novel techniques are being developed by many centers. By using advanced MRI sequences, supplemental information like functional or even metabolic information can be achieved besides anatomical images.
Dynamic contrast-enhanced magnetic resonance imaging(DCE-MRI) used a dynamic acquisition of T1-weighted MR images before, during, and after the administration of a contrast agent. A bolus of the appropriate contrast agent is injected into a vein, and rapid imaging techniques are then used to acquire serial images of a limited number of slices as the agent enters and leaves the tissue [87].
Contrast agents are usually gadolinium-based substances (Gd); nonetheless others such as superparamagnetic iron oxide (SPIO) have been tested [88]. These tend to shorten the T1 (Gd based) or T2 (SPIO based) relaxation times of protons causing measurable alterations in MR signal [64]. Some of the suggested uses of DCE-MRI are found in studying tumor microvascularization, lymphatics, and inflammation [64, 89].
Another new sequence that gradually is being incorporated into clinical routine is diffusion-weighted imaging (DWI) which is based on the restriction produced in the movement of water molecules in the interstitial space [90]. This is possible by detecting the apparent diffusion coefficient of tissue water (ADC). So in tissues with high cellularity, the movement of water molecules will be restricted showing low ADC, whereas tissues with low cellularity will show high ADC. DWI can be very useful when viable tissue is needed to be identified, for example, after an ischemic stroke, cancer imaging, or treatment response assessment [90, 91].
When needed functional information from MRI (fMRI), novel technique called blood oxygen level dependent (BOLD) has been introduced [92]. BOLD technique uses the endogenous deoxygenated hemoglobin to measure regional alterations, so higher levels of oxygenated blood will behave more intense on T2-weighted MRI images. It started mainly in neurology, to study brain functionality, cognition, memory, etc. [93, 94]. More clinical applications have been proven including the evaluation of differences in blood oxygen level in other tissues than brain and to evaluate the perfusion of tumors and response to treatment [92, 95, 96].
Magnetic resonance spectroscopy (MRS) cannot be considered a new technique because it has been used since 1950s [97–99], becoming an interesting element. MRS is based on applying electromagnetic pulses to a sample in an external magnetic field. Then, a tracer or probe is used to measure the results of the radiofrequency signals produced [64]. The inclusion of gradients that permits acquisition of positron data is the main difference between MRI and MSR. This is possible by creating a spectrum of peaks with the different chemical shifts, mainly lipids and metabolites, in a voxel of interest [64].
Commonly, MRS has been used for brain [100], breast, and prostate due to the good homogeneity of the magnetic field applied and the use of local acquisition coils with an outcome of a high signal-to-noise ratio. The spectral of metabolites used with MRS to study normal or abnormal physiology comprise choline, lactate, creatine, glutamate, lipids, and alanine.
To improve the sensitivity of MRS, a modified technique has been developed using a hyperpolarization enhancing the signal-to-noise ratio [101–103]. Hyperpolarized MRS has been performed with compound labeled with 13C [104]. One that has shown promise is 13C-pyruvate [85, 101] that plays a role in glycolysis and in preclinical studies such as 13C-urea for perfusion imaging [105] and 13C-fumarate for necrosis evaluation, among others [106]. For example, 13C-fumarate can also be used for prostate cancer [107, 108].
Last but not least, new classes of MRI contrast agents working selectively by reducing the magnetization of the water signal with a minimal effect on its longitudinal signal, instead of the proton relaxation-based approach, have emerged as promising tool showing interesting information. Chemical exchange saturation transfer (CEST) and paramagnetic-CEST (PARACEST) contrast agents provide specific molecular and physiological information in oncology, mainly pH sensing [109–113].
1.2.3 Radionuclide Imaging
Radionuclide imaging is probably one of the earliest and cultivated molecular imaging modality, including positron emission tomography (PET) and single-photon emission computed tomography (SPECT).
Radionuclide imaging uses radionuclides combined with other elements to form chemical compounds or else combined with existing pharmaceutical compounds, to form what is called radiopharmaceuticals.
Radiopharmaceuticals or radiotracers can target specific organs or cellular receptors (Fig. 1.5) enabling the evaluation of biochemical changes, cellular physiology, cellular function and metabolism, and levels of molecular targets in an individual.
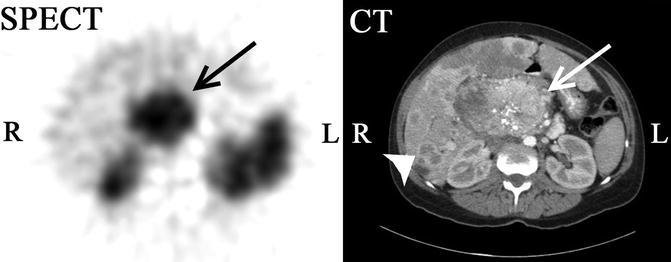
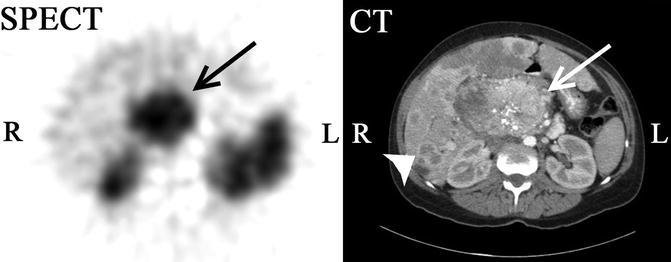
Fig. 1.5
111In-octreotide SPECT-CT scan of a 47-year-old female with a large abdominal mass. 111In-octreotide, a somatostatin analogue labeled with indium-111 binds somatostatin receptors which are overexpressed in some neuroendocrine tumors (NETs). The study shows an abdominal rounded mass (arrow) with intense tracer uptake demonstrating a tumor expressing somatostatin receptors. Contrast-enhanced CT evidences a central large heterogeneous mass with gross calcifications. In addition, multiple ring-enhancing liver lesions (arrowhead) consistent with metastasis can be seen (Courtesy of Dr. Pilar Bello, Department of Nuclear Medicine, University and Polytechnic Hospital La Fe, Valencia, Spain)
Through the years, advances in molecular imaging and radiochemistry have been used to develop new radiotracers or radiolabeled molecules to target different tissues, organs, or to even molecules rather than just anatomy.
Further, with the need of better anatomical information, hybrid modalities like SPECT/CT, SPECT/MRI, PET/CT (Fig. 1.6), and PET/MRI (Fig. 1.7) have been introduced into clinical practice offering not only molecular but also anatomical and functional information, all at once.
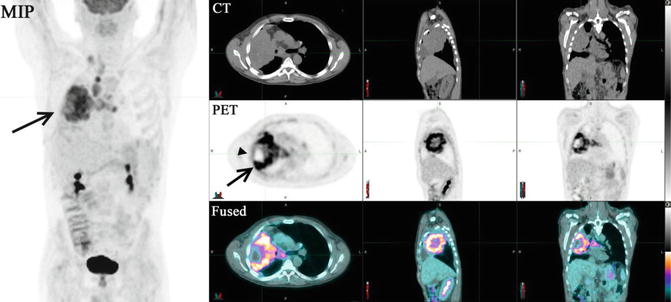
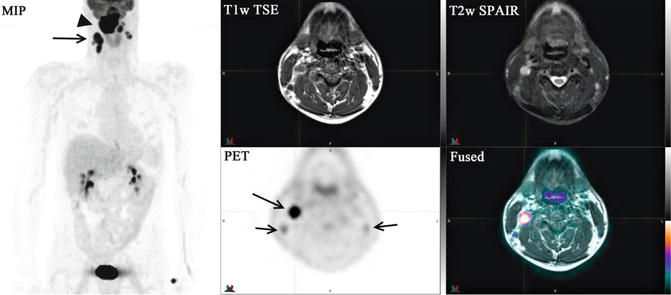
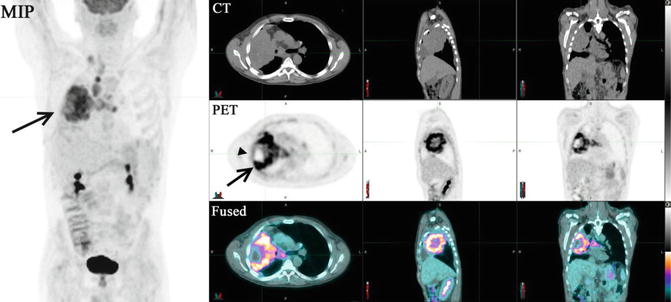
Fig. 1.6
18F-FDG-PET/CT (top row, CT; middle row, PET; bottom row, fused images) of a male with a recent diagnosis of small cell carcinoma of the lung. The PET study shows a highly intense heterogeneous abnormal tracer uptake (arrow) in the right mid-lung with several focal scattered areas of necrosis (arrowheads). The low-dose non-enhanced CT demonstrates a large rounded opacity occupying a large portion of the right lung extending contiguously into the right hilum with direct extension into adjacent lymph nodes. In addition, malignant intensity lymph nodes in the right supraclavicular fossa with extension into the precarinal and subcarinal space and anterior to the left bronchus are noticed
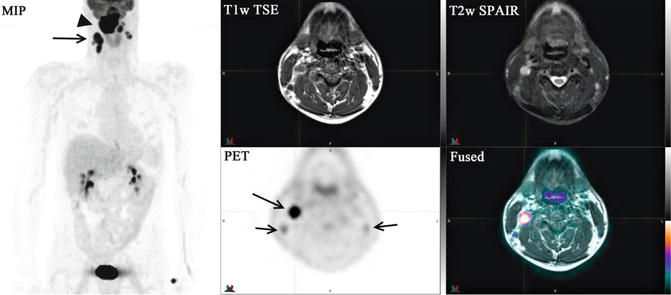
Fig. 1.7
18F-FDG PET/MRI (MIP image; top row, T1w TSE, T2w SPAIR; bottom row, PET, fused image) study of a 44-year-old male with a recently diagnosed invasive poorly differentiated squamous cell carcinoma of the nasopharynx. Large highly intense tracer uptake mass (arrowhead) involving the entire nasopharynx with extension to the skull base and involving the clivus which corresponds to the abnormal mass lesion identified in the MRI. Intense abnormal foci of tracer uptake are seen bilaterally in the posterior cervical region of the neck consistent with malignant lymphadenopathy (arrows)
There are many advantages of using radionuclide imaging among which is its high sensitivity, quantitative capabilities, limitless depth of penetration, and clinical utility. In addition, the innumerable possibility of targeting a specific molecule, receptor, antibody, drug, etc., makes radionuclide imaging a unique tool for clinical, preclinical, and research studies.
1.2.3.1 Single-Photon Emission Computed Tomography
The radionuclides used in single-photon emission computed tomography (SPECT) technology emit γ-rays during their decay. The most commonly used isotopes are 99mtechnetium (99mTc), 111indium (111In), and 123iodine (123I). They all have relatively long half-life, thus obviating the need of a cyclotron on-site [61]. The SPECT gamma camera scanner is composed of the scintillator, photomultiplier tubes, and collimator, which rotate around the patient through multiple angles and directions to capture data and obtaining a tomographic reconstruction. SPECT is more affordable and thus more extensively used that PET cameras. However, SPECT is less sensitive than PET because γ-ray photons don’t deliver adequate information regarding the origin of the photon, making impossible to define the line of response, as determined in PET [64].
Recent advances in improving sensitivity have been focused on high-resolution SPECT with pinhole scanners, different crystals used in the scintillators, etc. [114, 115]. Also, spatial resolution is lower than PET.
Hence, some disadvantages can be found on the ionizing radiation [116], like every RI modality, the limited spatial resolution already mentioned, and the lack of attenuation correction.
RI modalities such as SPECT or PET still lack spatial resolution compared with other imaging modalities so first, software fusion algorithm was developed to allow registration of functional and metabolic information gathered from RI with anatomical data from the CT or MRI, adding accuracy and precision to the studies [117]. As this has been expended, hybrid or multimodality systems have answered this demanding field by implementing CT or MRI components into the same gantry [118–121]. An additional benefit of combining SPECT with CT is the ability to be used not only for anatomical localization but also for attenuation correction [119, 122–124].
SPECT has limitless depth of penetration with an excellent sensitivity (though lower than PET), offering multiplexing capabilities. SPECT systems have the ability of distinguishing different radionuclides; therefore, it enables to image different targets simultaneously [125–127]. Furthermore, longer half-life period makes radiopharmaceuticals optimal for longitudinal studies.
Due to the enormous number of different radiopharmaceuticals designed for gamma cameras, it’s impossible to line them up; however some remarkable ones are listed in Table 1.1 [64, 128–131].
Table 1.1
SPECT radiopharmaceuticals (oncology related)
Radiopharmaceutical | Radiolabeling target | Applications |
---|---|---|
99mTc-MAA | Particles | Lung perfusion |
99mTc-DTPAaerosol | Particles | Lung ventilation |
99mTc-DTPA | Chelates | Glomerular filtration, blood brain barrier disruption |
Renal function | ||
Brain tumors | ||
99mTc-nanocolloid | Particles | Lymphatic drainage |
99mTc-sulfur colloid | Particles | Reticuloendothelial function (bone marrow, liver and spleen imaging) |
99mTc-MDP, 99mTc-HDP | Chelates | Bone formation |
99mTc-sestamibi | Chelates | Blood flow, tumor viability |
Myocardial perfusion | ||
Breast cancer, parathyroid, etc. | ||
67Ga-citrate | Chelates | Tumor viability, capillary leakage |
Tumor imaging | ||
Infection imaging | ||
99mTc-pertechnetate | As ions | Thyroid function |
123I-sodium iodine | As ions | Thyroid function |
131I-sodium iodine | As ions | Thyroid therapy |
111In-platelets | Cells | Cell incorporation (thrombus imaging) |
99mTc-red blood cellsdenaturated | Cells | Spleen imaging (accessory splenic tissue) |
111In-pentetrotide | Receptors | Somatostatin receptors (neuroendocrine tumors) |
123I-NP-59 | Receptors | Cholesterol metabolism (adrenal dysfunction) |
123 I-MIBG | Receptors | Presynaptic adrenergic receptors (myocardial failure) |
131I-MIBG | Receptors |