Fig. 1
a Large aneurysms (arrows) involving the proximal right and left coronary arteries in a 3 month old patient with Kawasaki disease obtained using a small field of view high resolution 3D cine TFE sequence with a two-element phased array coil. b In the same patient, a large field of view MR angiogram was performed with a cardiac phased array coil to demonstrate systemic involvement. Arrows point to aneurysms involving the right axillary and left iliac arteries. c Follow-up study 5 years later demonstrates complete resolution of aneurysmal changes in the right coronary artery. d High resolution imaging of the anomalous right coronary artery using a cardiac phased array coil on a 3T magnet, demonstrating the proximal intramural course (arrows)
2.4 Pulse Sequences
2.4.1 Black Blood Sequences
Conventional spin echo (CSE) T1-weighted sequence with ECG-triggering and respiratory compensation (Bailes et al. 1985) has traditionally been the mainstay for black blood imaging since the early days of cardiac MRI (Herfkens et al. 1983). This CSE sequence is no longer used primarily due to the long scan time. Acquisition time for spin echo sequences can be shortened by using segmented κ-space technique as in TSE or fast spin echo (FSE), by using echo planar imaging (EPI) readout, and by using parallel imaging techniques in combination. Parallel imaging allows for the use of higher number of signal averages (NSA) to compensate for respiratory motion (Chung and Muthupillai 2004) without significant increases in scan time. Respiratory triggering as a means of respiratory compensation can be used to reduce the number of signal averages needed, and in combination with thin sections of 2 mm or less, can be used to evaluate coarctation, vascular rings, vessel wall thickness, and airway compromise (Fig. 2). The general disadvantage with spin echo imaging is an incomplete “black blood” appearance with slow flow artifacts. Double or triple inversion recovery TSE sequence has been shown to produce excellent quality black blood images (Simonetti et al. 1996). However, breath-holding is necessary, which limits its utility in the pediatric population. This sequence can be used on freely-breathing patients with either multiple NSAs or with respiratory triggering, although the scan duration is longer. Clinically, this sequence is used when better nulling of the blood signal is needed, to minimize metallic artifacts from endovascular stents, and for myocardial tissue characterization to improve conspicuity of lesions such as cardiac tumors. A triple inversion recovery sequence provides additional fat suppression and can be used in edema imaging with T2-weighted sequences in acute myocarditis (Friedrich et al. 2009). With some modifications of this sequence, and in combination with respiratory navigator gating, black blood coronary artery imaging can be accomplished (Stuber et al. 2001). The dielectric shading artifacts on 3T MRI scanner has been minimized by multi-transmit radiofrequency (RF) technology such that reproducible diagnostic quality double inversion recovery black blood images can be produced routinely on 3T MRI scanners. (Mueller et al. 2012).
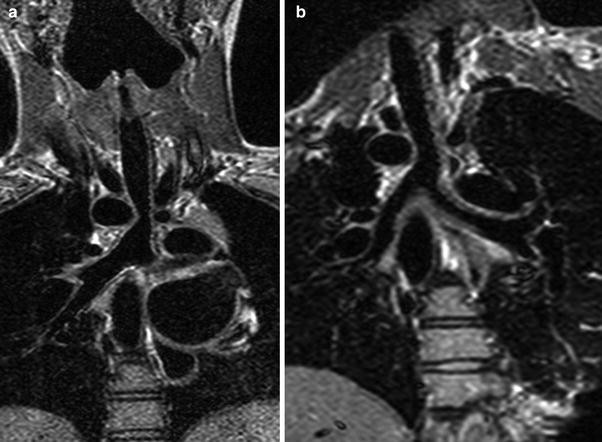
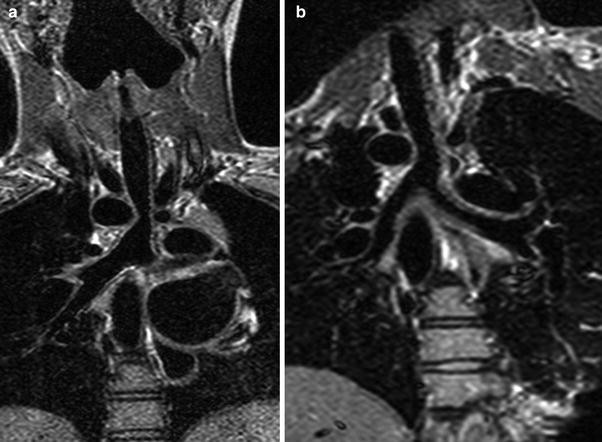
Fig. 2
Coronal black blood T1 echo planar images before (a) and after (b) surgery in a patient with double aortic arch with partial atresia of the left arch, demonstrating relief of airway compression after surgery
2.4.2 Bright Blood Sequences
2.4.2.1 Contrast-Enhanced MRI Angiography
For bright blood imaging, 3D contrast-enhanced magnetic resonance angiography (CE-MRA), initially introduced in the early 1990s (Prince et al. 1993), is the most efficient sequence for the evaluation of extra-cardiac vascular anatomy in the chest. The current implementation of this sequence is in the form of a 3D acquisition of T1-weighted fast gradient echo or turbo field echo (TFE) using a flip angle of 40–45°, and the shortest repetition time (TR) and echo time (TE) available on the scanner. This sequence is typically run with acquisition of multiple dynamic phases using gadolinium-based contrast agents injected intravenously. The early phases provide information about the pulmonary arterial tree, pulmonary veins, and the aorta and its branches, while the later phases provide information on systemic venous return. There is always a trade-off between spatial resolution and temporal resolution. In general, for the highest spatial resolution, respiration must be suspended and the arrival of contrast must be precisely timed to the vasculature of interest. But, by sacrificing some spatial resolution and using parallel imaging techniques, short dynamic scan times of 4–8 s can be achieved (Muthupillai et al. 2003; Chung and Krishnamurthy 2005). Therefore, in a young patient with high heart rates, who is sedated or cannot breath-hold, CE-MRA with parallel imaging offers an accurate and reproducible means of evaluating the extra-cardiac vasculature, with the rapid dynamics providing unsubtracted separation of the right heart, left heart, and systemic venous phases (Fig. 1b). The 3D volume rendered images provide an excellent overview of cardiovascular morphology (Fig. 3a) in cases with complex spatial relationships of the chambers. By combining with other rapid imaging strategies such as TRICKS (Grist and Thornton 2005) or CENTRA-keyhole-SENSE techniques (Beerbaum et al. 2006; Goo et al. 2007), CE-MRA can be achieved with temporal resolution of less than 2 s without compromise of spatial resolution. When the temporal resolution matches the respiratory cycle duration, the respiratory motion artifacts can be minimized during free-breathing acquisition (Krishnamurthy et al. 2010). Such high temporal and spatial resolution MRA techniques provide dynamic information on blood flow, parenchymal perfusion, shunting, and collateral pathways typically not available on CT angiography, and demonstrate diagnostic utility in a variety of pediatric conditions, including pulmonary artery stenosis, pulmonary vein stenosis, systemic vein obstruction, and arteriovenous malformations.
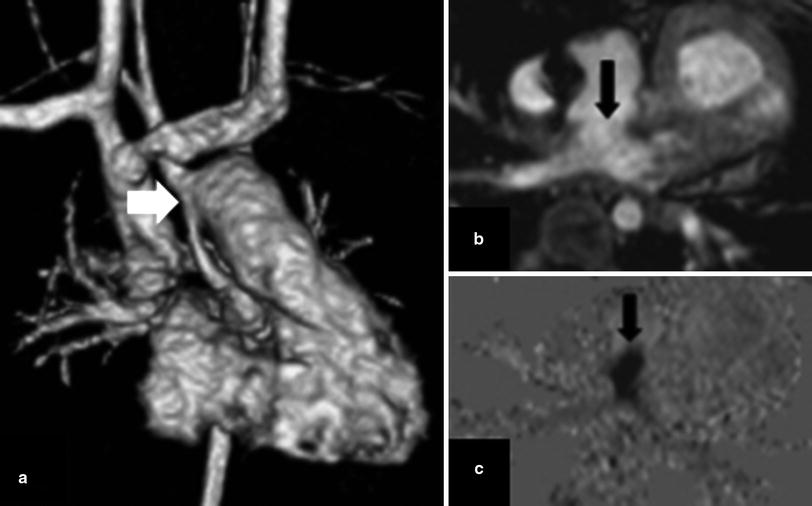
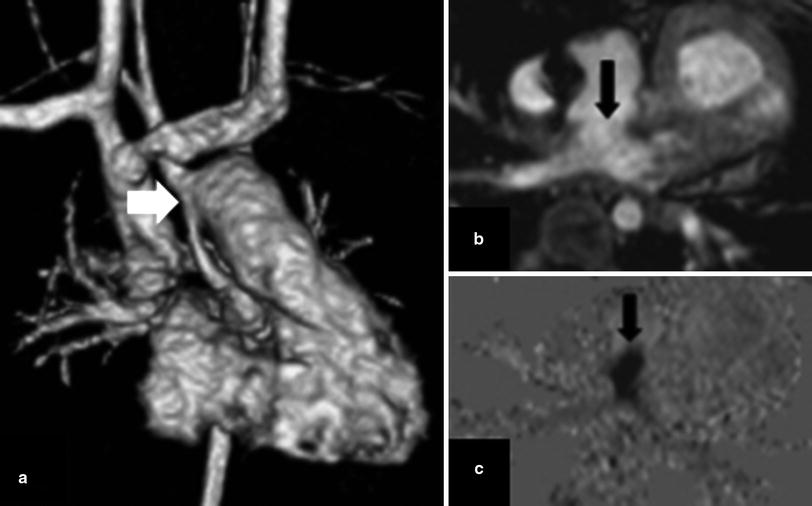
Fig. 3
Phase contrast imaging revealing restrictive atrial communication after a Norwood procedure for hypoplastic left heart syndrome. a 3D volume rendered image from an MRA shows changes of a Damus-Kaye-Stansel anastomosis between the main pulmonary artery and the hypoplastic ascending aorta (arrow). b Apparently patent atrial septostomy on the magnitude image (arrow). c Turbulent flow across septostomy demonstrated on the phase image, consistent with obstruction (arrow)
2.4.2.2 Cine Bright Blood Imaging
For cine bright blood imaging, ECG-triggered cine fast gradient echo or cine TFE with segmented k-space filling (Hernandez et al. 1993) and cine steady state free precession (SSFP) sequences are available. Despite the inherent flow related artifacts of the cine TFE sequence, this sequence is still preferred over SSFP for morphologic evaluation of extra-cardiac vascular pathology (Fig. 4), especially in smaller patients (Wood 2006). Higher spatial resolution can be achieved with cine TFE than with cine SSFP. Typically, 3 NSA with a SENSE reduction factor of 2 can yield adequate SNR on a cine TFE sequence. A saturation band placed over the anterior chest wall fat decreases ghosting artifacts related to breathing. Scan time will depend on the desired number of phases per cardiac cycle. In contrast, the cine SSFP sequence, known as true FISP (Free Induction Steady state Precession), bTFE (balanced Turbo Field Echo), or FIESTA (Fast Imaging Employing STeady state Acquisition) depending on the manufacturer, is acquired with breath-holding, and yields excellent quality images for intracardiac morphology and ventricular function (Fig. 5). When this sequence was first conceived in 1986 (Oppelt et al. 1986), the MRI hardware could not produce the short TR (<4 ms) and TE (<2 ms) necessary for the sequence to be clinically useful. Now, with advances in gradient strength, this is the sequence of choice for ventricular function evaluation due to its high temporal resolution, and excellent myocardial blood pool contrast (Carr et al. 2001). While using the cine SSFP sequence for ventricular function and intracardiac morphology evaluation, the aim is for a temporal resolution of 30–40 ms or approximately 20–25 phases per cardiac cycle with in-plane spatial resolution of 1.5–2.5 mm, depending on the strength and speed of the gradients of the MRI scanner. Parallel imaging is used with acceleration factor up to 2, whenever possible, to decrease the duration of breath-holding. Significant artifacts related to flow acceleration or pulsatile flow can be present in SSFP sequence despite careful shimming (Markl and Pelc 2004). To minimize artifacts, the lowest TR/TE is desirable, and there is a trade-off with spatial resolution. Cine TFE with segmented k-space filling may be a better choice for dynamic bright blood imaging in the setting of turbulent flow when higher spatial resolution is needed.
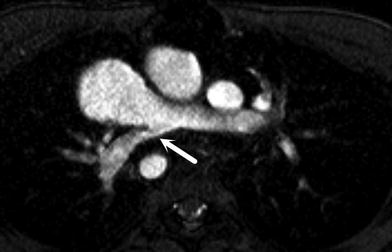
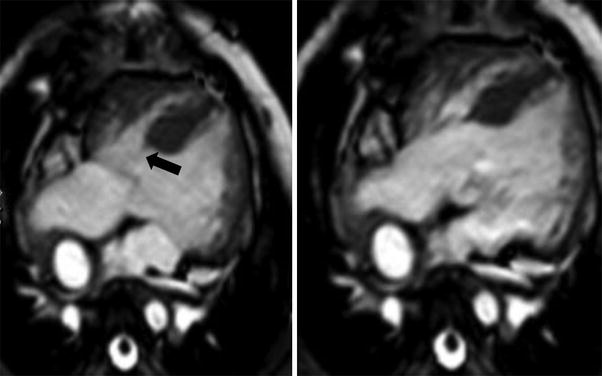
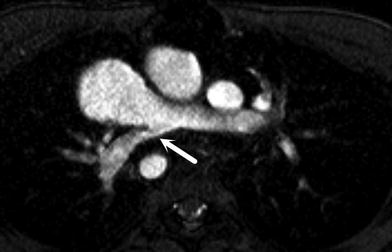
Fig. 4
Axial cine TFE image demonstrating high-grade stenosis of the origin of the right pulmonary artery (arrow)
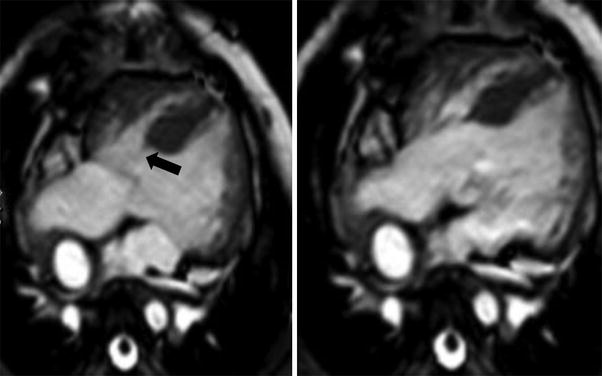
Fig. 5
Cine SSFP images in a patient with over-riding (valvular orifice over-riding the crest of the muscular ventricular septum) and straddling (arrow) (tension apparatus of the atrioventricular valve is attached in both ventricles) of the tricuspid valve in systole (left) and diastole (right)
For non-breath-hold cine SSFP scanning, multiple NSA can be used, but results are variable depending on the regularity of respiratory motion. The quality of the non-breath-hold scans in the short axis plane tends to be quite acceptable in the majority of patients, especially when they are sedated. Limited clinical experience has been reported with respiratory-triggered or navigator respiratory-gated free-breathing ventricular functional assessment using cine SSFP (Krishnamurthy et al. 2012; Atweh et al. 2013). Whether these new techniques can replicate or improve the excellent image quality associated with breath-held cine SSFP remains to be proven clinically, but they do offer the potential of free-breathing three-dimensional dynamic imaging of morphology and function of the entire chest in a single sequence. With the advent of multi-transmit radiofrequency technology in 3T MRI scanners, adequate diagnostic quality cine SSFP can be achieved routinely (Mueller et al. 2012; Chung 2012).
2.4.2.3 Coronary Imaging
Techniques of white-blood coronary artery imaging with respiratory navigator gating and fast gradient echo sequences (Kim et al. 2001; Flamm and Muthupillai 2004) are well developed for the evaluation of ischemic heart disease in adults. An isotropic whole-heart MRI examination with respiratory navigator gating and 3D SSFP has been used in adults to evaluate the coronary arteries (Weber et al. 2003; Sakuma et al. 2005), and has been applied to patients with congenital heart disease for global assessment of cardiac and extra-cardiac vascular anatomy (Sorensen et al. 2004). These techniques have also been used in the pediatric population in postoperative evaluation after coronary artery reimplantation, in patients with Kawasaki disease (Fig. 1), and in suspected coronary artery anomalies (Greil et al. 2002a, b; Su et al. 2004, 2005, 2007; Taylor et al. 2005a, b). Successful imaging with the higher heart rates encountered in children can be achieved with triggering to end-systole and shortening the data acquisition duration for every R–R interval (Beerbaum et al. 2009). With increasing availability of 3T clinical MRI scanners, high resolution (sub-mm in-plane resolution) coronary MRA can be achieved in selective applications such as demonstration of the intramural segment of anomalous coronary arteries (Fig. 1d). The whole-heart MRI examination has been recently enhanced by the use of the intravascular contrast agent, gadofosveset trisodium, in combination with an inversion recovery prepped SSFP or TFE sequence with inversion time of 260–280 ms (Makowski et al. 2011).
2.4.3 Flow Quantification
Noninvasive quantitative blood flow analysis is yet another powerful tool that MRI adds to the diagnostic armamentarium. The most widely used pulse sequence is a retrospective ECG-triggered cine phase contrast (PC), also known as velocity-encoded cine MRI (Brenner et al. 1992; Caputo et al. 1991; Helbing et al. 1996; Hundley et al. 1995; Powell and Geva 2000; Rebergen et al. 1993a, b, 1995; Sieverding et al. 1992; Steffens et al. 1994). The accuracy of this technique has been validated both in vitro and in vivo (Bogren et al. 1989; Evans et al. 1993; Firmin et al. 1987; Frayne et al. 1995; Kondo et al. 1991a, b; Powell et al. 2000; Greil et al. 2002a, b). Scan time can be shortened using segmented k-space technique, with the penalty of decreased temporal resolution (Kellenberger et al. 2005), and by combining parallel imaging techniques (Beerbaum et al. 2003; Prakash et al. 2006). Real-time phase contrast has also been applied clinically (Korperich et al. 2004). The main clinical applications include estimation of regurgitant fraction (Fig. 6) in patients with pulmonary regurgitation after repair of right ventricular outflow obstructive lesions such as tetralogy of Fallot (Helbing and de Roos 2000; Oosterhof et al. 2006), differential pulmonary flow to the right and left lungs (Roman et al. 2005; Sridharan et al. 2006), and systemic-to-pulmonary shunts (Powell and Geva 2000). Phase contrast techniques have also been used as a sensitive means of detecting flow dephasing to locate the presence of atrial and ventricular septal defects, to detect flow restrictive conditions (Fig. 3), and also for quantification of the gradient across a stenosis (Oshinski et al. 1996). The QP:QS is calculated by phase contrast imaging across the main pulmonary artery and ascending aorta, and provides an important decision-making tool in the presence of an atrial septal defect (ASD), ventricular septal defect (VSD), or anomalous pulmonary veins. 3D whole-heart volume acquisition of phase contrast data (4D flow) has been reported widely, although there are compromises related to decreased temporal resolution (Markl et al. 2011; Sorensen et al. 2005). More recently, exciting research work has been presented that combines compressed sensing on the acquisition side to speed up scan time and sophisticated post-processing tools to allow for simultaneous flow quantification, 3D flow visualization, and ventricular functional evaluation using the same 4D flow dataset (Hsiao et al. 2012). This has great potential to allow for operator-independent acquisition of volumetric data for congenital heart disease evaluation, and possibly even improve on flow quantification accuracy (Nordmeyer et al. 2013).
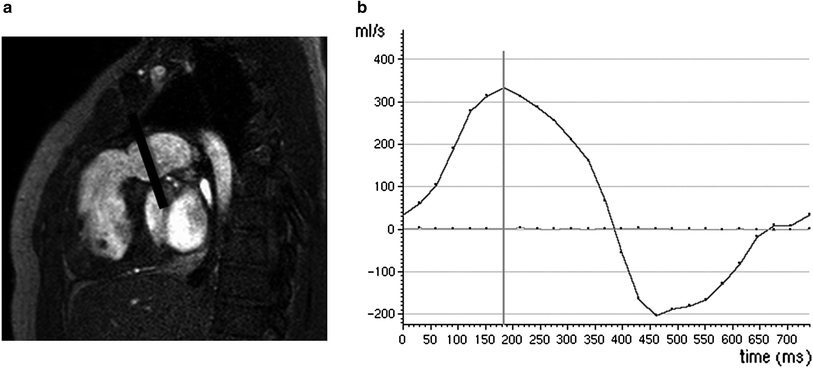
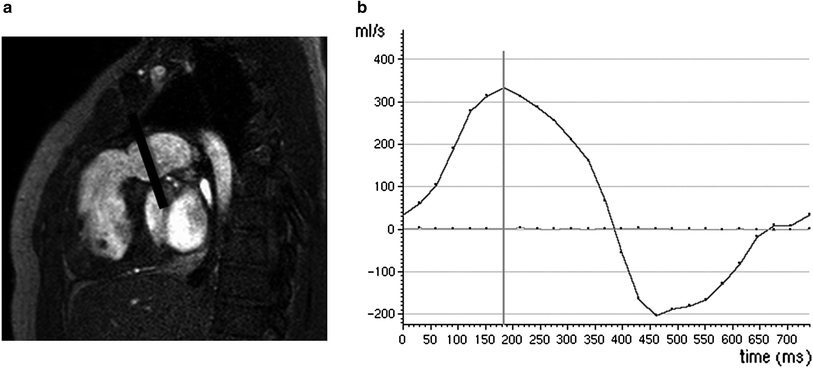
Fig. 6
a Cine TFE image in a 12-year-old patient with treated tetralogy of Fallot, showing a dilated right ventricular outflow tract due to severe pulmonary regurgitation, which can be quantified using a phase contrast sequence with a plane perpendicular to the RVOT (black line). b Quantitative flow volume curve across the RVOT reveals a stroke volume of 44 cc, and a regurgitant fraction of 45 %
2.4.4 Myocardial Function, Perfusion, Viability, and Tissue Characterization
Besides using cine SSFP, as previously described, for evaluation of global and regional myocardial function and wall motion, techniques for perfusion imaging (Schwitter 2006) with fast gradient echo (fGRE) or hybrid EPI-FGRE pulse sequences, (Jahnke et al. 2006), and viability imaging with an inversion recovery T1-weighted FGRE sequence (Kim et al. 2000) have been well established in adults for evaluation of ischemic heart disease. These techniques have also been applied to the pediatric population in the setting of treated congenital heart disease, especially after coronary reimplantation (Buechel et al. 2009a, b; Fratz et al. 2011), prolonged bypass procedures, tumors (Fig. 7), thrombus, right ventricular dysfunction, systemic right ventricle (Babu-Narayan et al. 2005; Fratz et al. 2006), single left ventricle (Rathod et al. 2010; Robinson et al. 2010), cardiomyopathy, myocarditis, and vasculitis (Prakash et al. 2004; Taylor et al. 2005a, b). With the widespread availability of phase-sensitive inversion recovery viability imaging (Kellman et al. 2002), robust viability imaging can be acquired in combination with respiratory navigator for sedated freely-breathing infants and young children. The acquisition can be performed either in end-systole or end-diastole. The right ventricular free wall can be more easily assessed in end-systole as the myocardium is thicker. An extension of this technique is quantitative T1-mapping of the myocardium, which may have potential for diagnosis of microscopic fibrosis in cardiomyopathy or myocarditis (Messroghli et al. 2007).
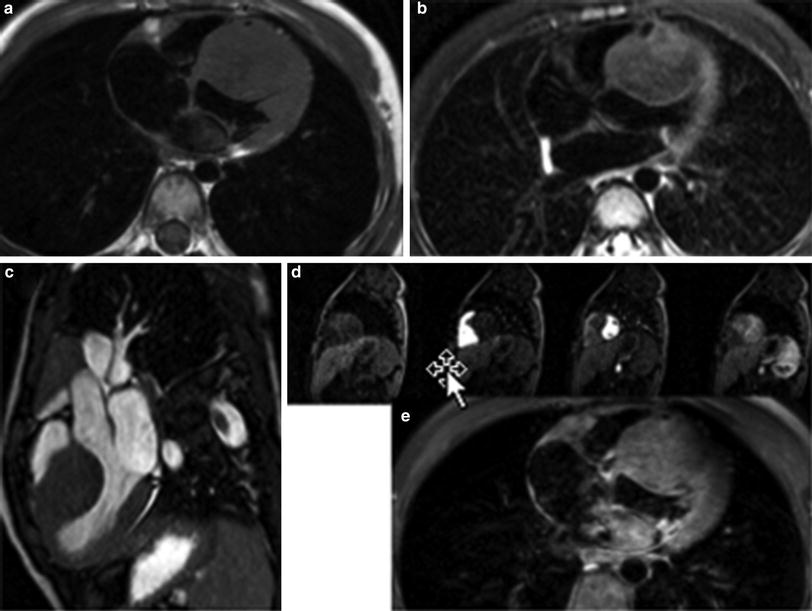
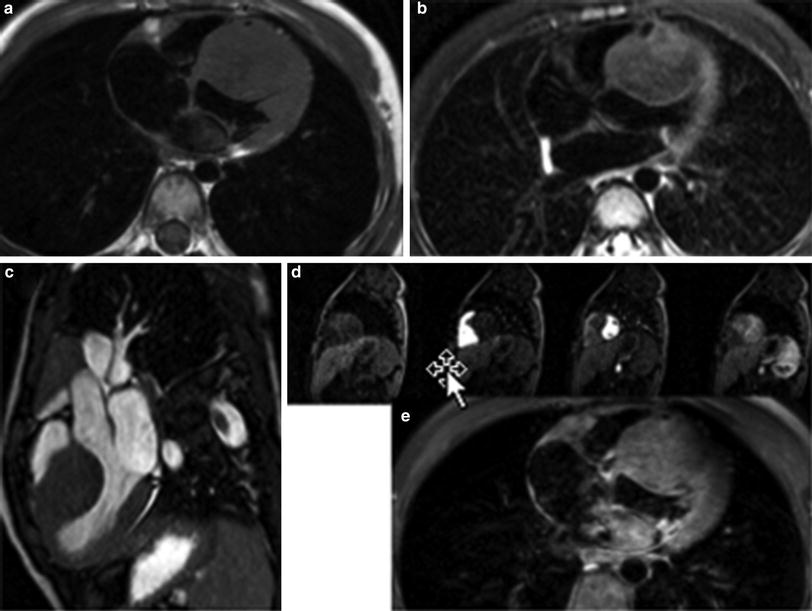
Fig. 7
Fibroma of the interventricular septum appearing isointense to myocardium on T1-weighted (a), and on T2-weighted (b) images, causing mild obstruction to LV outflow on cine SSFP (c), with perfusion (d) and enhancement (e) characteristics similar to myocardium after administration of gadolinium
Another technique of myocardial tissue characterization is T2* mapping. T2* mapping for iron quantification is a well-established clinical cardiac MRI examination in patients with thalassemia and sickle cell disease (Anderson et al. 2001; Westwood et al. 2005a, b; Wood 2009). Typically, a multi-echo fast gradient echo sequence with multiple TE’s is used and the T2* decay is measured in the septal wall after background noise correction (Westwood et al. 2003).
Myocardial tissue tagging was first applied clinically in the late 1980s (Zerhouni et al. 1988; Axel and Dougherty 1989). The basic principle is to “tag” the myocardium with either one-dimensional RF saturation lines or two-dimensional RF saturation grid at the beginning of a cardiac cycle. As the myocardium contracts, the “tag lines or grids” will deform. By analyzing the deformation of these tags, the mechanical properties of the myocardium such as stress and strain can be estimated. With the introduction of harmonic phase (HARP) MRI that allows for a faster and automated method of tagging analysis, there is more widespread application of tagging clinically (Castillo et al. 2005). On the hardware side, with 3T MRI, the tags persist much longer throughout the cardiac cycle compared to that on 1.5T, thereby improving the results of myocardial tagging (Valeti et al. 2006). It has been successfully applied in congenital heart disease (Fogel et al. 1998; Menteer et al. 2005), as well as in pediatric cardiology for the evaluation of cardiomyopathy due to Duchenne muscular dystrophy (Hor et al. 2009, 2011).
3 Evaluation of Extra-Cardiac Vasculature
MRI plays an important role in evaluation of the extra-cardiac vasculature in the preoperative and postoperative period. Evaluation of the systemic and pulmonary veins, branch pulmonary arteries, and the aorta by echocardiography is frequently limited by the lack of acoustic windows in older children, and in the postoperative setting. MRI provides various advantages over echo or CT in this situation, including a large field of view, arbitrary planes of evaluation, 3D imaging with high spatial resolution, excellent contrast resolution, excellent temporal resolution, freedom from artifacts related to surgical patch prostheses and calcification, the use of intravenous contrast with low nephrotoxic potential, and lack of ionizing radiation.
3.1 Aorta
3.1.1 Coarctation
MRI is a useful adjunct to echocardiography in the preoperative evaluation of coarctation, especially in the setting of suboptimal acoustic windows, or in atypical coarctation. In infancy and early childhood, echocardiography provides adequate information prior to surgery or balloon dilatation in discrete coarctation. But, if there is associated tubular hypoplasia of the aortic arch or atypical thoracic coarctation, then MRA or CTA help to define the extent of narrowing, the status of the head and neck arteries, as well as the collateral arterial supply, all of which are essential for surgical planning. In the postoperative period, echo windows diminished considerably, and MRA is preferred to CTA for serial follow-up due to the lack of ionizing radiation. The MRI protocol for recurrent coarctation comprises dynamic sequences for ventricular function, left ventricular outflow tract, as well as the aortic arch. The severity of the coarctation is determined by measuring the luminal caliber of the aorta, measuring the pressure gradient across the stenosis by flow velocity mapping of the aortic arch (Oshinski et al. 1996) (Fig. 8a), and by quantifying the amount of collateral arterial supply to the descending thoracic aorta by flow velocity mapping across the proximal and distal descending thoracic aorta (Steffens et al. 1994). Gadolinium-enhanced MRA demonstrates the location and extent of stenosis, the presence of pseudoaneurysms, the status of the head and neck arteries, as well as collateral arterial supply to the descending thoracic aorta (Fig. 8b).
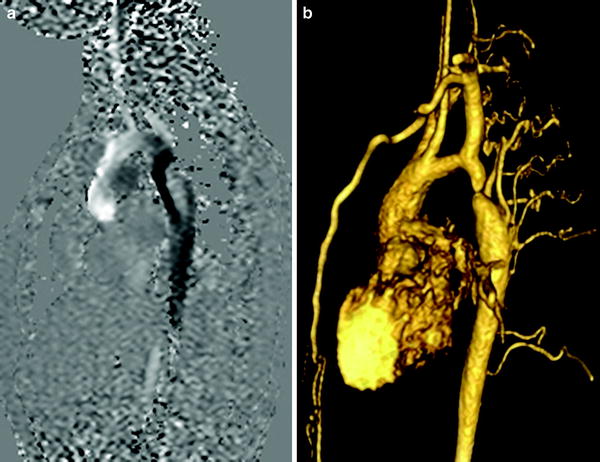
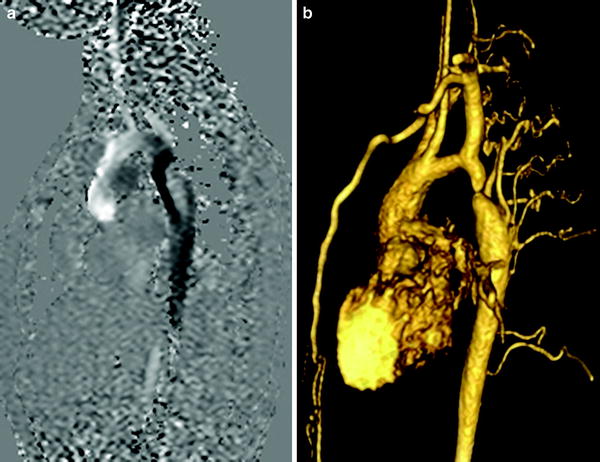
Fig. 8
a Flow velocity mapping of the aortic arch in a patient with coarctation showing a dephasing jet (arrow) distal to the stenosis with a peak velocity of 450 m/s. b Volume rendered image of a gadolinium-enhanced 3D MRA in the same patient showing the severity of stenosis, as well as the extent of collateral flow to the descending aorta
3.1.2 Aortic Root Dilatation
Patients with Marfan syndrome, Ehlers-Danlos syndrome, bicuspid aortic valve, or a history of a Ross procedure (replacing a dysplastic aortic valve with the native pulmonary valve) have a tendency to develop progressive aortic root dilatation (Grotenhuis et al. 2006). MRI provides an accurate, reproducible, and safe means of assessing aortic root caliber over time, which is essential to track stability or progression, and for decision-making regarding surgery. It overcomes the limitations of echocardiography, which is error-prone in the setting of eccentric aortic root dilatation. Ungated CT angiography is also limited in this location due to pulsation artifacts. The most helpful information is obtained from a stack of thin-section dynamic bright blood images performed perpendicular to the long axis of the aortic root and proximal ascending aorta, which allows calculation of maximal luminal caliber in systole and diastole, as well as extent of involvement (Fig. 9).
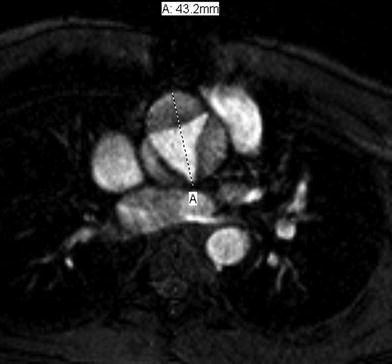
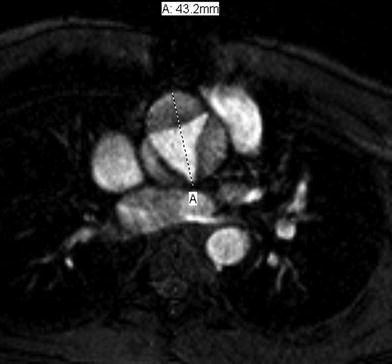
Fig. 9
Cine TFE image perpendicular to the aortic root in systole demonstrates severe aortic root dilatation in a 12 year old patient, who underwent a Ross procedure for sub-aortic stenosis
Flow velocity mapping across the ascending aorta is essential to calculate the aortic regurgitant fraction. In some cases, 3D coronary angiography is helpful to determine the status of the coronary origins prior to surgery, or in the postoperative setting.
3.1.3 Vascular Rings and Aortic Arch Anomalies
MRA provides an accurate and rapid means of determining the presence and nature of a vascular ring. Sequences include thin-section axial dynamic bright blood images through the upper thorax, and gadolinium-enhanced 3D MRA. High resolution black blood imaging is also performed to determine the status of the airway (Fig. 2). While the diagnoses of right arch with an aberrant left subclavian artery or double aortic arch are fairly straightforward, variations such as double aortic arch with partial atresia of the left arch may benefit from the dynamic nature of the MRI data when compared to CT angiography (Schlesinger et al. 2005). MRA is also accurate in diagnosing a number of other aortic arch anomalies including persistent fifth arch, interrupted aortic arch, and cervical aortic arch.
3.2 Pulmonary Artery
3.2.1 Pulmonary Sling
In a pulmonary sling, the left pulmonary artery arises anomalously from the right pulmonary artery, and travels between the trachea and esophagus to supply the left lung. There is associated compromise of the airway resulting from mass effect or coexisting tracheomalacia and bronchomalacia. Tracheal branching anomalies, including a T-shaped trachea, or complete cartilaginous tracheal rings, may also be present. The anomalous left pulmonary artery is frequently hypoplastic or has focal stenosis (Fig. 10). Using bright blood and black blood sequences as well as MRA, the entire spectrum of vascular and airway anomalies may be accurately depicted by MRI (Lee et al. 2001). Differential blood flow to the lungs may also be calculated using flow velocity mapping sequences.
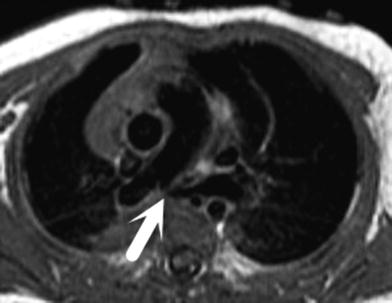
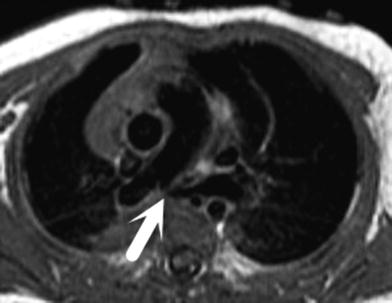
Fig. 10
Axial black blood fast spin echo double inversion recovery image demonstrating a pulmonary sling with high-grade stenosis of the proximal left pulmonary artery as it courses between the trachea and esophagus (arrow)
3.2.2 Branch Pulmonary Artery Stenosis
Branch pulmonary artery stenosis may be present as an isolated entity (Fig. 4), but is more commonly seen in the setting of Alagille syndrome, Williams syndrome, treated pulmonary atresia, tetralogy of Fallot, an arterial switch procedure for transposition of great arteries, or any condition involving RVOT conduit placement. The use of thin-section dynamic bright blood sequences, flow velocity mapping, and time-resolved contrast MRA successfully provides all clinically relevant information, including the severity and extent of stenosis, the pressure gradient across the stenosis, the status of the peripheral pulmonary vasculature, the differential pulmonary flow to both lungs, as well as the presence and location of aortopulmonary collaterals. The presence of associated pulmonary regurgitation or RV dysfunction may also be ascertained simultaneously using MRI. This is one of the more common indications for cardiac MRI in most pediatric practices.
3.3 Pulmonary Veins
3.3.1 Anomalous Pulmonary Veins
MRI is commonly used in the preoperative and postoperative setting for partial or total anomalous pulmonary venous return (Festa et al. 2006). In partial anomalous pulmonary venous return, which is associated with conditions like scimitar syndrome or sinus venosus defects, MRI is helpful in determining the presence and location of the pulmonary vein, the drainage area, the presence of associated obstruction, the degree of left to right shunting (QP:QS), and the presence of associated anomalies like pulmonary artery hypoplasia, aortopulmonary collaterals, and pulmonary airway malformations. MRI provides the most objective and reproducible data for surgical decision-making in partial anomalous pulmonary venous return. Most cases of total anomalous pulmonary venous return are successfully diagnosed by echocardiography in the newborn, with the use of MRI restricted to cases with complex anatomy like heterotaxy (Fig. 11), or in the setting of suboptimal echo windows. MRI has been successfully used to diagnose an unusual course of the aberrant pulmonary vein, as well as the presence of associated stenosis, both in infradiaphragmatic and supradiaphragmatic TAPVR.
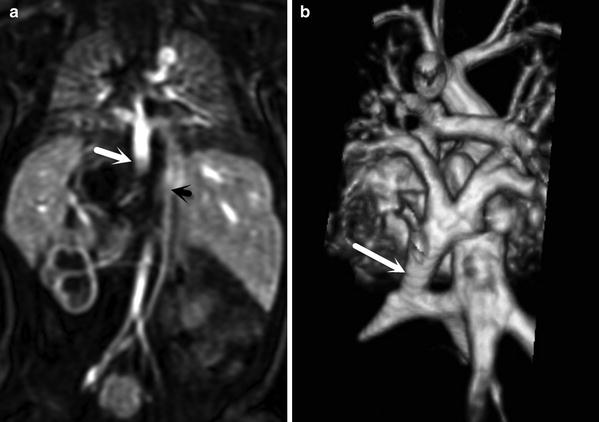
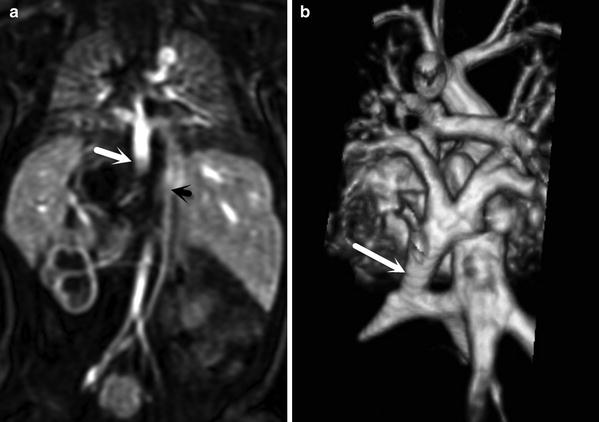
Fig. 11
MIP (a) and volume rendered (b) images of an MRA in a newborn with heterotaxy and right isomerism, showing total anomalous pulmonary venous return below the diaphragm to a hepatic vein (large white arrow), a left sided IVC (small black arrow), a transverse liver with asplenia, and a right-sided stomach
3.3.2 Pulmonary Vein Stenosis
Pulmonary vein stenosis may occur as an isolated entity, or after repair of anomalous pulmonary venous return or other types of congenital heart surgery (Fig. 12), and is usually difficult to manage with a poor prognosis. MRI determines the presence, severity, and extent of stenosis, as well as its impact on pulmonary arterial supply, differential pulmonary blood flow, and ventricular function (Valsangiocomo et al. 2003). One major limitation of MRI in this setting is its inability to determine pulmonary vascular resistance and right ventricular chamber pressures, although estimates may be obtained from pulmonary artery caliber and interventricular septal motion.
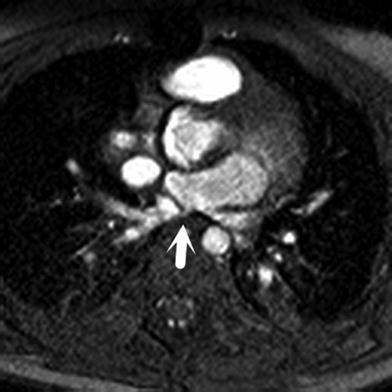
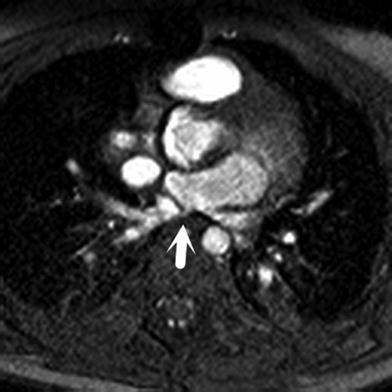
Fig. 12
Recurrent severe pulmonary vein stenosis (arrow) after repair of total anomalous pulmonary venous return
3.4 Systemic Veins
Systemic venous anomalies frequently coexist with congenital heart disease, especially in the setting of heterotaxy (Fig. 11). The most common anomaly is a persistent left superior vena cava, which drains into the coronary sinus. A connecting vein between the two superior vena cavae is typically absent in this setting. Interruption of the IVC with azygos continuation, and/or aberrant hepatic venous drainage is frequently present in heterotaxy. These anomalies significantly alter surgical management in single ventricle repair, in which the cavopulmonary connections have to be modified to accommodate the aberrant venous drainage.
Systemic venous obstruction is common after congenital heart surgery or prolonged central vascular catheter usage, and MR venography (MRV) offers an accurate means of diagnosing the extent of thrombosis, the acuity of the process, and the degree and adequacy of collateral venous flow. Simultaneous imaging of the brain should be considered in the setting of SVC or bilateral jugular vein thrombosis to assess for elevated intracranial pressure, which may manifest with ventriculomegaly or intracranial hemorrhage.
4 Evaluation of Cardiac Morphology
The segmental approach to the diagnosis of congenital heart disease (Van Praagh 1984) includes the following analyses:
1. What is the anatomic type of the three major cardiac segments: the viscero–atrial situs, the ventricles, and the great arteries? Consider the example of a patient with complete transposition of the great arteries (d-TGA) with VSD and pulmonary stenosis. The cardiac segments are: situs solitus of the atria and viscera, D-looping of the ventricles (morphologic right ventricle lies to the right of the morphologic left ventricle), and D-malposition of the great arteries (aortic valve annulus lies anterior and to the right of the pulmonary annulus).
2. How is each segment connected to the adjacent segment? In the example of d-TGA described above, there is atrioventricular concordance and ventriculoarterial discordance.
3. What are the associated malformations? In the example described above, the patient also has a conoventricular VSD and pulmonary valve stenosis associated with d-TGA.
4. How do the segmental combinations and connections, along with the associated malformations, function? The patient with the morphology described above will have cyanosis and reduced pulmonary blood flow.
4.1 Clarifying Complex Segmental Cardiac Anatomy
Viscero-atrial situs abnormalities and cardiac malpositions are usually easily identified by echo. However, in the presence of situs ambiguous, atrioventricular or ventriculoarterial discordance, or anomalous pulmonary or systemic venous connections (Fig. 11), difficulties may arise in defining the topographic relation of the major cardiac segments (Araoz et al. 2002). MRI is an excellent technique for defining the morphologic features of each atrium and ventricle. MRI depicts anatomical details that are easily related to the surrounding structures of the body, and thus provides reliable diagnoses in heterotaxy (Sorensen et al. 2004; Kersting-Sommerhoff et al. 1990a, b). In patients with complex anomalies, especially in older patients, MRI may be the primary imaging technique so as to maximize noninvasive information prior to catheterization.
4.2 Atrial Pathology
The sensitivity of echo for diagnosing secundum-type ASD is close to 100 % in infancy, but drops to 85–90 % in older children and adults. Transesophageal echocardiography (TEE) is a trusted method of sizing an ASD prior to surgery or percutaneous device closure, but is invasive, uncomfortable, and may carry a small risk of morbidity and mortality. MRI may be a useful noninvasive alternative in patients who refuse or are unable to tolerate TEE and may provide additional information on the shape and location of the ASD. ASD sizing by MRI using bFFE and phase contrast (PC) protocols correlates well with TEE estimations, and PC-MRI provides additional information on ASD shape and proximity to adjacent structures (Piaw et al. 2006). MRI guidance has also been used to navigate endovascular catheters and deliver ASD closure devices (Henk et al. 2005).
The superior sinus venosus defect is the most difficult form of atrial septal defect to detect echocardiographically due to the extreme rightward and superior position of this type of defect. The inferior type is also difficult to depict by transthoracic echocardiography because of its infero-posterior location relative to the fossa ovalis. MRI has become the gold standard for depiction of sinus venosus defects (Fig. 13). The coronary sinus septal defect involves partial absence of the atrial septum between the coronary sinus and the left atrium due to incomplete development of the left atrio-venous fold. It may be associated with left SVC to coronary sinus, unroofing of the coronary sinus, and other complex congenital heart defects, and its evaluation by MRI can overcome the limitations of echocardiography. MRI may also identify ASD or partial anomalous pulmonary venous connection in adults with right-sided chamber enlargement, hypertrophy, or dysfunction of unknown etiology. In all forms of septal defects, quantification of shunt size (pulmonary to systemic flow ratio, otherwise known as the Qp:Qs) by flow velocity mapping compares favorably to other imaging techniques, and enables decision-making regarding conservative therapy versus surgery (Hundley et al. 1995).
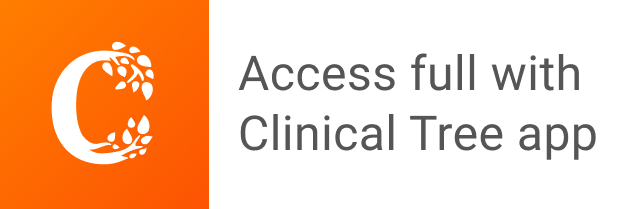