Although currently not approved by the US Food and Drug Administration (FDA) for cardiac imaging, the vast majority of cardiovascular magnetic resonance (CMR) studies use a gadolinium-based contrast agent. The CMR contrast agent typically makes diseased tissue appear brighter (or in some cases darker) than the surrounding tissue. Cardiovascular applications, such as magnetic resonance angiography (MRA), functional imaging of myocardial perfusion, and viability with late gadolinium enhancement (LGE) imaging of fibrosis represent the bulk of CMR scans that use a contrast agent. The first magnetic resonance (MR) approved contrast agent, gadopentetate (Gd-DTPA), appeared in 1988, and several other compounds followed. These first contrast agents were extracellular fluid (ECF) agents. Although frequently used in CMR and an essential component of CMR perfusion and LGE assessment of fibrosis, none of these agents is currently approved by the FDA for cardiac applications. Thus, the use of these agents in CMR is considered “off-label.” There are now also an approved hepatobiliary contrast agent and an intravascular agent designed specifically to enhance contrast-enhanced (CE) MRA. At the preclinical stage, there are exciting advancements in molecular imaging agents, including compounds that detect pH changes, enzymatic activity, specific biomolecules such as fibrin or collagen, and magnetically labeled cells.
This chapter focuses first on the general underlying chemistry and biophysics of contrast agents in clinical CMR. The mechanism of action of different classes of contrast agents is described, with examples drawn from CMR applications. Finally, there is a brief survey of novel contrast agents potentially useful for cardiovascular indications that are currently in clinical or preclinical development.
The vast majority of CMR agents in clinical use are small molecules based on chelated gadolinium (Gd). The bulk of this chapter focuses on gadolinium complexes, including their chemistry, biophysics, and applications. Other exogenous compounds have been used to change signal properties in MRI (e.g., iron particles, hyperpolarized nuclei), and these will be discussed as appropriate to CMR. This chapter assumes that the reader has a basic understanding of CMR vocabulary, and the reader is referred to Chapter 1 for further clarification.
Introduction to the Biophysics of Magnetic Resonance Imaging
All contrast agents shorten both T1 and T2 relaxation times. However, it is useful to classify CMR contrast agents into two broad groups based on whether the substance increases the transverse relaxation rate (1/T2) by roughly the same amount that it increases the longitudinal relaxation rate (1/T1) or whether 1/T2 is altered to a much greater extent. The first category is referred to as “T1 agents” because, on a percentage basis, these agents alter 1/T1 of tissue more than 1/T2 as a result of endogenous transverse relaxation in tissue. With most pulse sequences, this dominant T1-lowering effect gives rise to increases in signal intensity, and thus these agents are referred to as “positive” contrast agents. The T2 agents largely increase the 1/T2 of tissue selectively and cause a reduction in signal intensity, and thus they are known as “negative” contrast agents. Paramagnetic gadolinium-based contrast agents are examples of T1 agents, whereas ferromagnetic large iron oxide particles are examples of T2 agents.
There are many mechanisms by which contrast agents shorten T1 and T2. Considerable chemistry and biophysics can be applied to understand or predict these mechanisms. However, in many cases, the effect of these mechanisms can be reduced to a single constant, called “relaxivity.” Fig. 3.1 shows the effect and definition of relaxivity.
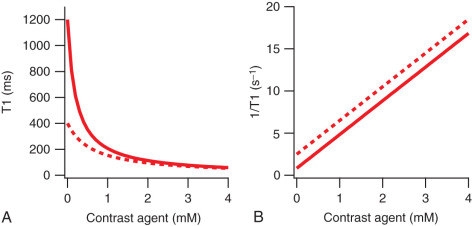
Fig. 3.1A shows the effect of a typical contrast agent on the relaxation time of two hypothetical tissues, one with T1 = 1200 ms (similar to heart muscle at 1.5 T) and one with T1 = 400 ms. At low concentration (left side of the graph), it appears that the contrast agent has a larger effect (change in T1) on the tissue with the longer T1. At higher contrast agent concentrations (right side of Fig. 3.1A ), both tissues approach approximately the same T1. A simple way to quantify this effect is to consider the rate of relaxation, 1/T1 (sometimes denoted “R1”). In most cases in medical imaging, the contrast agent increases the relaxation rate proportional to the amount of contrast agent:
1T1=1T10+r1[CA]
1T1=1T20+r2[CA]
Contrast agent behavior in vivo is seldom as simple as the pure linear effect relaxation rate shown in Fig. 3.1 . Even in the simple case of pure linear relaxation, the effect of the contrast agent on the CMR image is generally nonlinear. In traditional spin echo sequences, nonlinearity can be a result of T1 saturation or T2 signal loss. Once the contrast agent reduces T1 < repetition time (TR)/2, increasing contrast agent concentration will have little effect on increasing the available longitudinal magnetization because the tissue will have nearly fully recovered the magnetization before the next radiofrequency (RF) pulse. Furthermore, because contrast agents affect both T1 and T2 relaxation, at high enough concentration, the contrast agent will reduce T2 to the order of the echo time (TE), and will then decrease MR image intensity. These effects are seen in Fig. 3.2 , where signal intensity is plotted versus contrast agent concentration for T1- and T2-weighted spin echo sequences. Fig. 3.2 was generated assuming a contrast agent relaxivity of 4 mM −1 s −1 , typical of most commercial ECF gadolinium agents, and tissue relaxation times typical of myocardium at 1.5 T (T1 = 1200 ms, T2 = 50 ms). For the T1-weighted sequence (TE/TR = 15/600), Fig. 3.2A , signal intensity begins to level out at a contrast agent concentration between 0.5 and 1.0 mM. From Fig. 3.1A , this is the range at which the T1 of the myocardium decreased to approximately 300 ms, or TR/2. At concentrations >1 mM, the T1 effect is saturated, and the only imaging effect of the contrast agent is to make T2 shorter and cause signal loss, even on this T1-weighted sequence. Signal is lost because even a T1-weighted sequence has a finite TE, and T2 effects can enter when T2 is short enough.
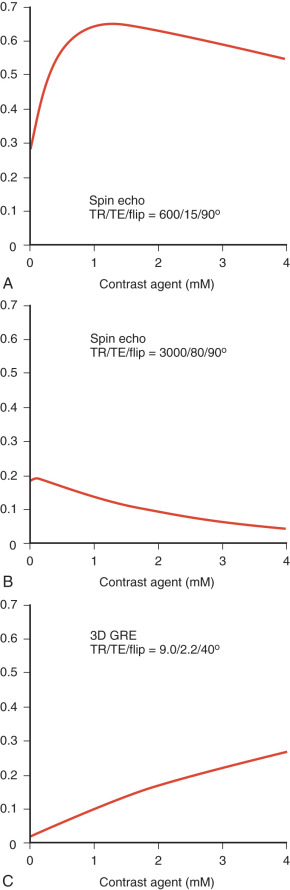
The signal intensity plateau on the T2-weighted scan (TE/TR = 80/3000), Fig. 3.2B , occurs at much lower contrast agent concentration. Because TR is so long, the only real effect of the contrast agent is to reduce (rather than increase) signal intensity on this T2-weighted scan. However, this negative contrast can also be medically useful, and certain contrast agents (notably, the iron oxide particles) create negative contrast exactly by providing enhanced T2 relaxation, and thus darker images on T2-weighted scans.
Increasing the relaxivity ( r 1 or r 2 ) will have the effect of pushing the simulated curves in Fig. 3.2A to the left, which means that peak signal and subsequent signal loss will occur at lower contrast agent concentrations. A more linear response of signal to contrast agent can be achieved with a fast three-dimensional (3D) spoiled gradient recalled echo (GRE) sequence. This is seen in Fig. 3.2C , where signal intensity is plotted versus contrast agent concentration using the same tissue relaxation times and relaxivities as in Fig. 3.2A and B for a typical fast 3D spoiled GRE sequence, TE/TR/flip = 2.2/9.0/40 degrees. The short TR and very short TE ensure that signal intensity increases across the entire concentration range. At high concentration, the effect of the contrast agent is becoming nonlinear, but the signal is still increasing with increasing contrast agent concentration.
Commercial Contrast Agents and Those in Clinical Development
The addition of paramagnetic materials to reduce relaxation times goes back to the earliest days of MR. In the 1940s, Bloch and colleagues used ferric nitrate to enhance the relaxation rate of water. Exogenous contrast was applied to MRI in 1978 when Lauterbur and associates reported using manganese dichloride to differentiate normal from infarcted myocardium in dogs. Carr and colleagues reported the first use of a gadolinium complex, gadopentetate dimeglumine (Gd-DTPA; Magnevist, Bayer, Berlin, Germany), in patients with brain tumors in 1984. By 1988, Gd-DTPA was FDA approved for clinical use.
Extracellular Agents
The most common contrast agents used clinically are ECF agents. Although several are approved for clinical use, as mentioned previously, none is specifically FDA approved for cardiac applications. These all behave in a very similar manner, and are typically referred to as “gadolinium” or “gado” agents. Fig. 3.3 shows the chemical structures of several approved ECF agents. Chemically, these compounds exhibit three similar features: they all contain Gd, they all contain an 8-coordinate ligand binding to Gd, and they all contain a single water molecule coordination site to Gd. Nomenclature for contrast agents can be confusing: there is a generic name (e.g., gadopentetate dimeglumine), a trade name (e.g., Magnevist), and usually a chemical code name or abbreviation (e.g., Gd-diethylenetriaminepentaacetic acid or Gd-DTPA). Any of these three names may be used in the scientific literature.
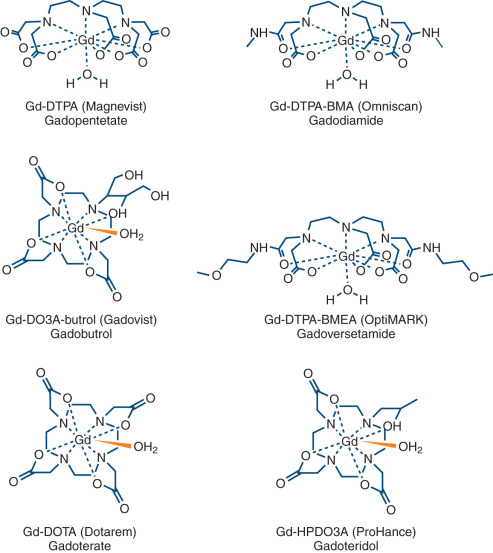
The multidentate ligand is required for safety. The ligand encapsulates the Gd, resulting in a high thermodynamic stability and kinetic inertness with respect to metal loss. This enables the contrast agent to be excreted intact, an important property because these contrast agents tend to be much less toxic than their substituents. For example, the DTPA ligand and gadolinium chloride both have an LD 50 of 0.5 mmol/kg in rats (LD 50 = dose that causes death in 50% of the animals), whereas the Gd-DTPA complex has a safety margin that is higher by nearly a factor of 20, with an LD 50 of 8 mmol/kg for the Gd-DTPA complex.
The Gd ion and coordinated water molecule are essential to providing contrast. The Gd (III) ion has a high magnetic moment and a relatively slow electronic relaxation rate, properties that make it an excellent relaxer of water protons. The proximity of the coordinated water molecule leads to efficient relaxation. The coordinated water molecule is in rapid chemical exchange (10 6 exchanges/s) with solvating water molecules. This rapid exchange leads to a catalytic effect whereby the Gd complex effectively shortens the relaxation times of the bulk solution.
The ECF agents have very similar properties, and these are summarized in Table 3.1 . They are all very hydrophilic complexes with similar relaxivities and excellent safety profiles. In addition, they can be formulated at high concentrations. On injection, these ECF agents quickly and freely distribute to the extracellular space. Administration of any of these agents (with rare exceptions) yields similar diagnostic information.
Generic Name | Product Name | Chemical Abbreviation | r 1 , 0.47 T 40°C | r 2 , 0.47 T 40°C | Osmolality a (Osmol/kg) | Viscosity a (cP) |
---|---|---|---|---|---|---|
Gadopentetate | Magnevist (Bayer HealthCare Pharmaceuticals) | Gd-DTPA | 3.4 | 4.0 | 1.96 | 2.9 |
Gadoterate | Dotarem (Guerbet Group) | Gd-DOTA | 3.4 | 4.1 | 1.35 (4.02) | 2.0 |
Gadodiamide | Omniscan (GE Healthcare) | Gd-DTPA-BMA | 3.5 | 3.8 | 0.79 (1.90) | 3.9 |
Gadoteridol | ProHance (Bracco Diagnostics, Inc.) | Gd-HPDO3A | 3.1 | 3.7 | 0.63 1.91 | 1.3 (3.9) |
Gadobutrol | Gadovist (Bayer HealthCare, Inc.) | Gd-DO3A-butrol | 3.7 | 5.1 | 0.57 (1.39) | 1.4 (3.7) |
Gadoversetamide | OptiMARK (Mallinckrodt, Inc.) | GD-DTPA-BMEA | 4.2 | 5.2 | 1.11 | 2.0 |
Gadobenate | Multihance (Bracco Diagnostics, Inc.) | Gd-BOPTA | 4.2 | 4.8 | 1.10 | 5.3mPas |
Gadofosveset | Ablavar (Lantheus Medical, Inc.) | MS-325 | 5.8 | 6.7 | 0.83 | 3.0 |
Gadoxetic acid | Eovist (Bayer HealthCare Pharmaceuticals) | Gd-EOB-DTPA | 5.3 | 6.2 | 0.69 | 1.2 |
a All concentrations 0.5 M except those in parentheses, which are 1 M.
There are some differences among the physical properties. The diamide complexes gadodiamide and gadoversetamide have considerably lower thermodynamic stability (log K ~ 17 vs. log K > 21 for other Gd complexes). The nonionic (neutral) compounds gadodiamide, gadoteridol, gadoversetamide, and gadobutrol were designed to minimize the osmolality of the formulation. This was prompted by the distinct reduction in toxicity and side effects brought on by the development of nonionic x-ray contrast media. However, for CMR, the injection volumes are much smaller than the volumes used for x-ray angiography or computed tomography (CT) angiography. Thus the overall increase in osmolality after injection of a CMR contrast agent is minimal. Unlike with x-ray contrast, there is no documented safety benefit in using nonionic CMR contrast agents. One benefit of the nonionic compounds is the ability to formulate them at high concentration (1 M) without drastically increasing osmolality or viscosity (see Table 3.1 ). These high-concentration formulations may be useful in fast dynamic studies, such as dynamic MRA or myocardial perfusion. These ECF agents, as with iodinated preparations used for x-ray and CT, are excreted by the kidneys. As a result, clearance is impaired in patients with impaired/reduced renal function (discussed later).
Blood Pool Agents
Because CMR contrast agents are administered intravenously, they are all potentially capable of imaging the blood lumen. The ECF agents described earlier are used routinely for CE-MRA (see Chapter 25, Chapter 26, Chapter 44, Chapter 45, Chapter 46 ). One potential drawback of using ECF agents for MRA is their pharmacokinetics. ECF agents rapidly leak out of the vascular space into all the interstitial spaces of the body. Angiography with ECF agents is thus typically limited to dynamic/first-pass arterial studies. There has been considerable effort made toward designing specific blood pool agents that would be tailored for vascular imaging. The ideal blood pool agent should remain in the vascular compartment and not leak out into the extracellular space. It should be capable of being given as a bolus such that a dynamic arterial image can be obtained. At the same time, it should have sufficient relaxivity and blood half-life to allow high-resolution steady-state images to be obtained. MS-325 (gadofosveset, initially marketed as Vasovist; and more recently as Ablavar; in the United States by Lantheus Medical Imaging, Billerica, MA; Fig. 3.4 ) is approved, and there are several other blood pool agents that have reached various stages of clinical development. However, as this chapter was being written, Lantheus announced that it would no longer produce gadofosveset. Three approaches have been taken to design blood pool agents: protein binding, increased size, and ultrasmall iron oxide particles. These are discussed later.
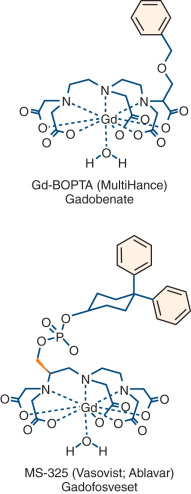
MS-325 (gadofosveset) is a Gd-based compound that binds reversibly to serum albumin. Albumin is the most abundant protein in plasma, and its concentration is high enough (600–700 µM) to reversibly bind most of the MS-325 after injection. Reversible albumin binding serves four purposes: (1) the albumin slows the leakage of the contrast agent out of the intravascular space; (2) the reversible binding still allows a path for excretion—the unbound fraction can be filtered through the kidneys or taken up by hepatocytes; (3) the bound fraction is “hidden” from the liver and kidneys, leading to an extended plasma half-life; and (4) the relaxivity of the contrast agent is increased 4-fold to 10-fold on binding to albumin (discussed later). Gd-BOPTA (gadobenate; see Fig. 3.4 ) has weak affinity for albumin (~10% bound), which leads to a modest increase in relaxivity relative to the other ECF agents.
The binding and relaxivity features of Gd-based blood pool agents are listed in Table 3.2 . Because binding affinity is moderate to weak for these compounds, the fraction bound to albumin will depend on the concentrations of albumin and the contrast agent. Immediately after injection, when the concentration of the contrast agent is high relative to albumin, there will be a greater free fraction. As the concentration of the contrast agent begins to stabilize (at ~0.5 mM) the fraction bound will become constant. The observed relaxivity will depend on the fraction bound; unlike ECF agents, the plasma T1 change is not linearly related to contrast agent concentration.
MS-325 Gadofosveset | Gd-BOPTA Gadobenate | Gadomer-17 a | |
---|---|---|---|
Agent type | Strong protein binding | Weak protein binding | Increased size |
r 1 buffer (mM −1 s −1 ) | 6.6 | 4.4 | 16.5 |
r 1 plasma (mM −1 s −1 ) | 50 | 9.7 | 19.0 |
% bound plasma | 91 |
Early work on blood pool compounds involved Gd covalently linked to macromolecules, such as polylysine, dextran, or modified bovine serum albumin. The large size of these compounds restricted diffusion out of the vascular space and led to very good vascular imaging properties. However, these compounds cleared very slowly in preclinical studies and there were concerns about a potential immunologic response. An alternative approach is to use a coated iron oxide nanoparticle. So-called ultrasmall superparamagnetic particles of iron oxide (USPIO) are very large (10–30 nm) but still small enough to evade substantial capture by the reticuloendothelial system. Such particles also have strong r 1 relaxivity, especially at lower field strengths. Unlike the Gd-based compounds, which will extravasate to some extent, these iron oxide particles are true intravascular blood pool agents. Currently there are no USPIO commercially available that are approved for MRI, but ferumoxytol (Feraheme) is a carboxymethyldextran-coated USPIO that is approved as an intravenous iron replacement therapy. Ferumoxytol has been used off-label as a blood pool agent and has seen some use in pediatric CMR owing to the long circulating blood half-life which facilitates high-resolution imaging and obviates the need for timing of boluses. Ferumoxytol can also be used to image monocytes and macrophages on delayed phase imaging (next day) as accumulation in the reticuloendothelial system is the ultimate fate of these particles. The iron oxide particles are not excreted; the iron is eventually resorbed into the body.
The Guerbet company is developing a new extracellular fluid agent with high relaxivity. The compound P03277 is reported to have relaxivities of 12.8 mM −1 s −1 at 1.5 T and 11.6 mM −1 s −1 at 3 T. This Gd chelate contains two water molecules bound to the metal ion, which leads to the high observed relaxivity. P03277 is a low molecular weight (970 Da) contrast agent that distributes in the extracellular space similarly to other ECF agents and has blood elimination kinetics comparable to gadobutrol in animal models. At the time of writing (2017), P03277 is currently undergoing Phase 2 clinical trials.
Relaxivity
Because their effect is indirect, CMR contrast agents differ from other diagnostic imaging agents. Water and fat are imaged, and it is the action of the contrast agent on the relaxation properties of the water hydrogen nuclei that generates contrast; in x-ray contrast media and nuclear imaging agents, the effect observed is more direct. Because water is present at a very high concentration (55,000 mM) and the contrast agent is typically at a much lower concentration (0.1–1 mM), the contrast agent must act catalytically to relax the water protons for there to be a measurable effect. Relaxivity, r 1 and r 2 , thus describes this catalytic efficiency. Some compounds are better magnetic catalysts than others (have higher relaxivity). Moreover, relaxivity is dependent on the external magnetic field, B 0 . This section explains these differences and the molecular basis for them. For discrete ions, such as Gd(III) and manganese (Mn[II]), the factors influencing relaxivity are the same; for iron oxide particles, the relaxation mechanism is different and will be treated separately.
Relaxivity can be factored into inner- and outer-sphere terms. “Inner sphere”
r1=Δ(1/T1)[Gd]=r1IS+r1OS
r1IS=q/[H2O]T1m+τm
Inner-sphere relaxivity is given by Eq. 3.4 , where q represents the number of water molecules bound to the metal ion (typically q = 1), [H 2 O] is the water concentration in millimolars, T 1m is the relaxation time of the water that is bound to the metal ion, and τ m is the lifetime of the water molecule in the inner sphere. Eq. 3.4 shows that the more sites available for water to bind ( q ), the more efficient the process. Likewise, the T1 of the bound water (T 1m ) should be very short. This is indicative of how effectively the metal ion can relax the coordinated water. The lifetime τ m of the bound water is the inverse of the water exchange rate ( k ex = 1/τ m ). If turnover is slow (τ m is long), then it will not matter how efficiently the bound water is relaxed if this relaxation cannot be transmitted to the bulk water.
Paramagnetic relaxation (1/T 1m ) occurs via a dipolar mechanism. At a magnetic field encountered in medical imaging, 1/T 1m is shown in Eq. 3.5 , where C is a constant,
1T1m=CS(S+1)r6MH[3τc1+ωH2τc2]
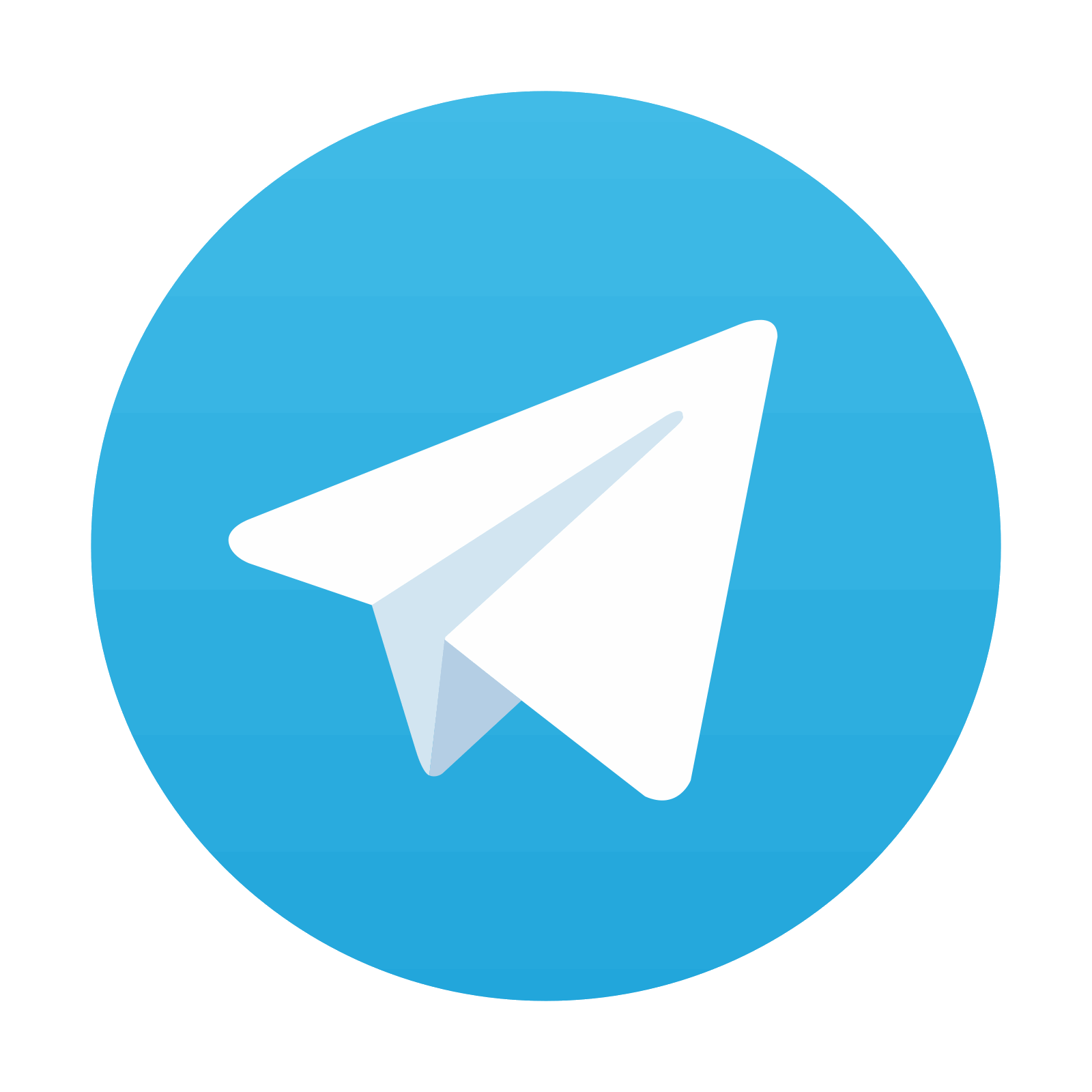
Stay updated, free articles. Join our Telegram channel
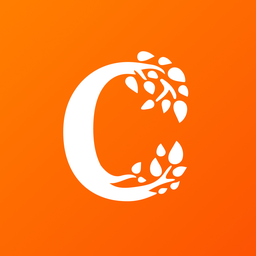
Full access? Get Clinical Tree
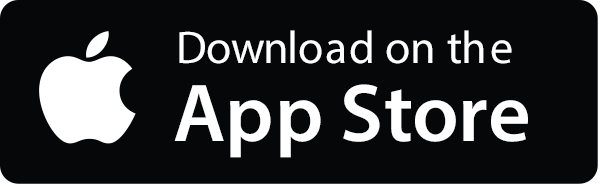
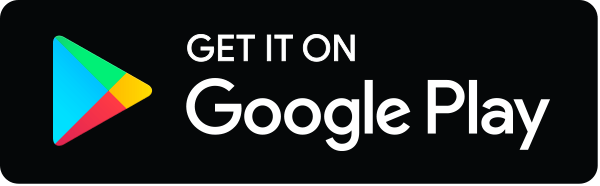
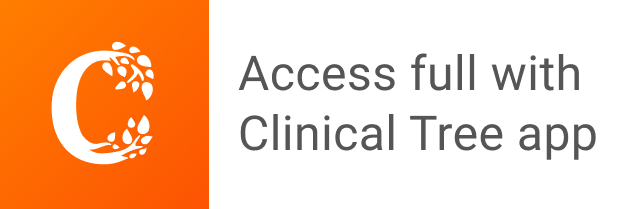