5 Computed Tomography The prognostic significance of coronary artery calcium has been recognized for many years, but the application of cardiac computed tomography (CT) first enabled a reproducible, quantitative determination of coronary calcium. CT can detect calcifications and define their spatial extent. The quantification of coronary artery calcium is an important issue because a definite relationship exists between the extent of coronary atherosclerosis and the extent of coronary calcium. More coronary calcium is associated with a greater risk of cardiovascular events such as myocardial infarction and cardiac death (Fig. 5.1). Conversely, the absence of coronary calcium correlates with a very low cardiovascular risk, at least in the 45- to 74-year age group. Based on these relationships, coronary calcium determination has been incorporated into the guidelines for cardiovascular risk assessment of the major professional societies in Europe and the United States.1–3 To make an accurate coronary calcium determination consistent with published guidelines, it is important to understand the technical capabilities and limitations of CT. Despite efforts of users and manufacturers to establish uniform standards, differences in the algorithms used for quantification of coronary calcium and the use of different types and generations of CT scanners have made it difficult to compare calcium determinations published by different authors. This should be considered in the interpretation and reporting of coronary calcium findings. Coronary calcium is a largely specific expression of coronary atherosclerosis. Because of the correlation between the extent of calcification and the extent of atherosclerosis, the accurate quantitative determination of coronary calcium is an important concern. Electron-beam computed tomography (EBCT) was conceived in the late 1970s and introduced clinically in the early 1980s. The first cardiac CT scanners had image acquisition times of 50–100 ms per slice.4 In 1990, Agatston et al. published a proposed algorithm for the quantitative determination of coronary artery calcium.5 This algorithm was later called the Agatston score (Fig. 5.3). Although based on EBCT, it still has major importance today because almost all data on the prognostic implications of coronary artery calcium have been acquired using EBCT technology and the Agatston score. EBCT differs from modern CT in that it employs a greater distance between the cathode and anode, approximately 3 m. This design eliminates mechanical motion of the X-ray tube. The only rotating component is the electron beam that generates the X-rays. The electron beam is electromagnetically steered to sweep in a 210° range across the anode, called the target ring. The sudden deceleration of the electrons (as in every CT scanner) results in the emission of X-rays, which emerge from the anode beneath the patient, pass through the patient’s body, and are registered by a detector array linked to a digital measuring system. Fig. 5.1 Meta-analysis of four studies on calcium scores and cardiovascular risks. A total of 3970 asymptomatic patients were followed for an average of 3.6 years (13 000 person years) (after Pletcher5a). Fig. 5.2 Calculation of the Agatston score. A CT density > 130 HU is assumed to indicate a calcified lesion. The arrow indicates a lesion of the proximal left anterior descending artery. The size of this lesion and its maximum CT density enter into the Agatston score. One advantage of EBCT over modern CT scanners is lower radiation exposure, which is in the range 0.5–1.0 mSv. For many years the very short image acquisition time was a unique feature of EBCT and has recently been equaled only by up-to-date CT units. The single-slice scanning technique of EBCT is a disadvantage, however, as it requires a long breath hold that may exceed 30–40s depending on the number of slices and the patient’s heart rate. Additionally, the abrupt movements of the scanner table necessary for contiguous slices are a potential source of motion artifacts. On the whole, EBCT has not become widely utilized despite its groundbreaking cardiac applications and still short acquisition time, due primarily to its high costs and limited clinical applications. The Agatston calcium score, introduced in 1990, still has an important role owing to its proven prognostic significance. This is all the more remarkable when we consider that the score was instituted on an arbitrary basis without scientific foundation. For purposes of Agatston scoring, a calcification is defined as an area of increased CT density measured in Hounsfield units (HU, named for the CT pioneer Sir Geoffrey Hounsfield). The CT density of water is 0 HU, and the density of air is – 1000 HU. Coronary calcifications cause greater X-ray attenuation, leading to values considerably greater than 0 (up to ~600). The Agatston score defines the lower threshold for calcifications as a value > 2 SD above the mean density of blood, corresponding to 130 HU. The area of the lesion is calculated in mm2 based on the number of contiguous pixels (numbering at least 2) that show calcific density. Additionally, the lesion is assigned a “density factor” of 1–4 based on its maximum calculated CT density. The density factor is 1 if the CT density is between 130 and 199 HU, 2 for a CT density between 200 and 299 HU, 3 for a density between 300 and 399 HU, and 4 for a density of 400 HU or more. The Agatston calcium score is a dimensionless quantity defined as the product of lesion area times density factor (Fig. 5.2). The lesions distributed throughout the coronary tree are added together to yield a total score. Experimental validation studies have shown that, despite the arbitrary criteria used in the Agatston calcium score, the score shows good agreement with the true extent of vascular calcification as measured by histomorphometry or weight after 3 days’ heat treatment.6 Nevertheless, the Agatston calcium score does not always have good reproducibility, especially in small lesions. This is due mainly to ambiguities inherent in the density factor. If the maximum density of a lesion is measured at 198 HU, the density factor is equal to 1. If the maximum density is measured at 201 HU, the density factor is 2. Assuming a lesion area of 8 mm2, the calcium score would be 8 in the first case and 16 in the second case. Several studies on the interscan variability of the Agatston calcium score suggest that the score should be at least 30–50 to achieve acceptable interscan variability. When the Agatston calcium score is determined with a multislice CT scanner (MSCT), the difference in imaging technology must be taken into account. Modern CT scanners all have a thinner slice thickness than the traditional 3-mm slice thickness of EBCT. Accordingly, comparable protocols must be applied to transfer the Agatston score to these scanners. The calcium scores determined with a 4-slice CT scanner appear to correlate quite well with the calcium scores determined by EBCT.7,8 However, protocol selection and slice thickness are crucial. Given these circumstances, the determination of coronary calcium mass is becoming an increasingly important test as it is largely independent of the type of scanner used. Electron-beam computed tomography was first used specifically for cardiac CT imaging and established the principle of coronary calcium determination based on the Agatston score. This quantitative coronary calcium score is the basis for most sectional and prognostic studies on the importance of coronary calcium determination. The volume calcium score was specially developed to allow a more precise calcium score determination in progression studies. This score does not employ a density factor. As in the Agatston calcium score, a CT density higher than 130 HU is considered to indicate calcium. As the name suggests, the volume score is obtained by determining the volume of the calcified lesion. Isotropic interpolation of the original data is used in an effort to achieve greater accuracy.9 Using the original dataset, measurements are taken at various points within the slice corresponding to the size of the pixels in the cross-sectional plane (e. g., 0.51 mm in the 26-cm2 field of view of traditional EBCT). The density values are assumed to have a linear distribution so that the interpolated voxels can be calculated. These calculations are performed automatically with software currently available. Although the volume score has been successfully used in several progression studies, its advantages over the Agatston score appear to be small. Comparative studies have not shown that the volume score is more reproducible than the Agatston score.10 As a result, application of the volume score has basically been limited to scientific progression studies. Calcium mass can be determined accurately and reproducibly by scanning a calibrated phantom that contains cylinders with varying, precisely defined calcium masses. In this way a calibration factor can be determined and the calcium mass can be measured in milligrams. Today this analysis is generally included in the processing software of modern MSCT scanners. Comparative studies have shown that calcium mass determination remains very stable across different imaging protocols, slice thicknesses, and acquisition times, while the Agatston calcium score may show considerable variation.11 The calcium mass in milligrams measured in the coronary tree is in a ratio of ~1: 4.5 to the dimensionless Agatston score, depending on the image acquisition protocol. Determination of the coronary calcium mass will assume greater importance in the future because it provides internationally comparable measurements that are independent of CT technology and image acquisition protocols. Differences in the quantification of coronary calcium relating to different scanners and algorithms can largely be avoided by using an algorithm calibrated to calcium masses measured in a phantom. Given this advantage, calcium mass determination is assuming an increasingly important role. Modern MSCT technology produces a true volumetric dataset, as the continuous rotation of the X-ray tubes yields overlapping datasets that can be processed in thin slices.12 This reduces partial volume effects and the slices can be reduced to a submillimeter thickness, resulting in true isotropic resolution. Additionally, data can be acquired continuously throughout the cardiac cycle, making it possible to reconstruct images at various points in time and eliminate motion artifacts during certain phases of the cardiac cycle. The radiation dose can be reduced by “tube current modulation,” i. e., periodic lowering of the tube current during cardiac cycle phases in which imaging data are not acquired (Fig. 5.3). Meanwhile, MSCT imaging permits the prospective triggering of image acquisition at a predefined point in the cardiac cycle. The radiation dose is in the range 1.0–3.0 mSv, depending on the particular scanner and protocol. Regarding procedural recommendations on coronary calcium determination by MSCT, the importance of the shortest possible acquisition time and a slice thickness of 3 mm or less should be emphasized.13 The currently available multislice scanners (usually 16 slices or more) offer sufficient image quality for this purpose. Recommendations differ regarding the prospective ECG triggering of image acquisition and the retrospective ECG gating of acquired images that is used in most spiral scanners. The advantages of spiral scanning (true volumetric dataset, variable timing of image reconstructions) result in better reproducibility and thus greater validity of the findings, but spiral scans also expose the patient to a somewhat higher radiation dose. Both protocols (retrospective gating, prospective triggering) have been used successfully in the Multi-Ethnic Study of Atherosclerosis.14 The image quality of modern MSCT scanners permits the accurate quantification of coronary calcium. With continuous spiral scanning, image acquisition can be timed to any point in the cardiac cycle by the retrospective selection of sectional images for analysis (retrospective gating). Another option is prospective ECG triggering, in which data are acquired at a predefined point in the cardiac cycle as in EBCT. Continuous spiral scanning yields the best image quality, and prospective triggering provides the best reduction in radiation dose. As in CT coronary angiography, when the calcium score is determined by spiral CT the examination should be followed by suitable data reconstructions to minimize artifacts that could distort the calcium score. For example, motion artifacts from calcified plaques may cause blurring of the calcifications in systolic reconstructions (smearing or blurring artifacts). Various phenomena may be observed, depending on the size of the calcified plaques. The density of small plaques may fall below the 130 HU threshold owing to blurring artifacts, with the result that the analytic software no longer recognizes them as calcified lesions (Fig. 5.4). Motion artifacts also affect the quantification of large calcified plaques. Blurring artifacts in this case lead to overestimation of the actual score. The degree of overestimation depends on the amplitude of the motion. Selection of the reconstruction interval in retrospective gating can influence coronary calcium determination (over- or underestimating the actual amount of calcium). This effect is less pronounced in the latest generation of scanners than in older instruments. Distortion of the calcium score due to artifacts is especially likely to occur in patients with a very high heart rate. It is best to examine patients with a heart rate of ~60 bpm. If necessary, β-blockers should be given orally or intravenously before the test to lower the heart rate to the target range. Caution is urged in patients who have coronary stents or have undergone coronary bypass surgery, because stents and clips cannot always be distinguished from coronary calcium, resulting in overestimation of the calcium score. The accuracy of calcium score determination is limited in both groups of patients. Also, scanning conditions are often compromised in patients with cardiac pacemakers, and artifacts from the pacemaker leads can be particularly troublesome in evaluations of the right coronary artery. Fig. 5.3 Principle of multislice spiral CT. Continuous table feed moves the patient through the rotating beam, which simultaneously scans multiple slices within the body. The acquisition of image information occurs at designated times in the cardiac cycle, typically within the end diastole. These image acquisition times are indicated by black rectangles and broken-line ovals in the diagram. The radiation is reduced between acquisitions. Fig. 5.4a, b Images of the cardiac base (64-slice MSCT). The image reconstructed at 55% of the R–R interval shows a calcified lesion in the proximal left anterior descending artery (arrow in a). However, image reconstruction at 70% of the R–R interval results in a density below the cutoff value of 130 HU and the lesion is no longer depicted as a calcification (arrow in b). Table 5.1 reviews typical image acquisition protocols with retrospective gating for multislice CT scanners in current use. A standard scheme should be followed in the reporting of coronary calcium findings. The examination itself must conform to the minimum requirements and quality criteria noted above to produce a meaningful report. We recommend the following 9-point scheme in reporting the findings of a coronary calcium examination: 1. Give clinical information regarding the patient’s symptoms, prior cardiac history, and risk factors. 2. State the basic clinical question (e. g., “risk stratification”). 3. Give methodological details with regard to scanning technology (EBCT, MSCT), manufacturer, scanner type, and calcium mass determination and a brief description of the calibration method. 4. Indicate basic scan parameters with regard to slice pattern (contiguous, overlapping), slice thickness, coverage (scan volume should always cover the entire coronary system), slice acquisition with prospective or retrospective ECG gating, and any deviations from the recommended tube current (mAs) and voltage (kV). 5. Describe the image quality as good or degraded owing to artifacts (respiratory artifacts, extrasystoles). Indicate “noise” in the aorta (state mean density and standard deviation in the aortic root). 6. Indicate the method of coronary calcium quantification (Agatston calcium score, volume calcium score, calcium mass) for the entire coronary system and separately for the left main stem, left anterior descending artery, left circumflex coronary artery, and right coronary artery. 7. Evaluate extracoronary cardiac calcifications and aortic calcium. 8. Indicate age and sex percentiles, and classify the finding by stating the source (own series or drawn from the literature). 9. Make a risk assessment based on coronary calcium quantification and percentiles. Although rapid advances in CT technology with changes in hardware, image acquisition protocols, and analytical software pose an obstacle to uniform measurements at the present time, there has still been a marked overall improvement in the image quality and reproducibility of coronary calcium determination. Besides technical advances, this stems partly from the efforts of users to establish comparable protocols as well as standard interpretation and reporting schemes. The determination of coronary calcium mass has an important role in this regard, and there has been steady progress toward more reproducible examinations with increasingly shorter image acquisition times and thinner slices.15 The Agatston calcium score will continue to be important, as it is the measure upon which current prognostic data and percentiles in various groups of selected and unselected patients are based. The basic principle of computed tomography (CT) is that a narrow X-ray beam passes through the patient’s body from various directions. The attenuation of the X-ray beam is measured along each of the beam paths by detectors, and a mathematical process (filtered back projection) is used to calculate the attenuation coefficient at each point in the scanned section. The relative attenuation values are expressed in Hounsfield units (HU) and represented as a gray-scale image. The Hounsfield scale ranges from – 1000 HU for air through 0 HU for water and has no upper limit (it is ~1500 HU for compact bone). CT angiography of the coronary vessels can be performed with electron beam CT (EBCT) or with a multislice spiral CT (MSCT) system. In “conventional” CT, the X-ray tube moves around the patient. In EBCT, however, the electrons generated in an electron gun are aimed at a tungsten target, and the resulting X-ray emissions are directed through the patient to the detector rings. In theory, EBCT is capable of demonstrating the larger, proximal coronary arteries, but there are major practical limitations such as relatively low spatial resolution, dose limits, high cost, and limited availability. For these reasons, CT angiography of the coronary vessels is performed almost exclusively with multislice (multidetector) spiral CT systems. There are two basic requirements for CT imaging of the heart: • High temporal resolution to prevent motion artifacts • High spatial resolution to define even small anatomical structures like the coronary arteries This has become possible through rapid advances in CT technology during the past 15 years. The revolution in CT began in 1991 with the introduction of spiral CT. Until then, CT scanning was a stop-and-shoot technique in which the patient table was advanced a short distance, paused for the acquisition of a single slice, then advanced again. Even with a large slice thickness of 5–10 mm, a complete thoracic examination took 5–10 minutes and required numerous breath holds. Spiral CT, on the other hand, acquires image data during continuous table motion, enabling the entire thorax to be scanned as a 3D volume in a single breath hold. Meanwhile the rotation time of the X-ray tube has been dramatically shortened. In 1999, a CT system with four detector rows and a gantry rotation time of 500 ms was introduced that allowed ECG-triggered imaging of the heart. Scanners with 16 detector rows and a gantry rotation time of 420 ms were introduced two years later, making it possible for the first time to make a detailed evaluation of the heart and coronary arteries. Systems with 64 detector rows and gantry rotation times as short as 330 ms have been available since mid-2004. By end of 2007 initial results of 256-detector-row CT have been published and recently a 320-slice CT has been launched. Whereas scanners with more detector rows are able to improve coverage per rotation and spatial resolution the introduction of dual-source computed tomography (DSCT) in 2006 has significantly improved the temporal resolution of ECG-gated multidetector-row cardiac computed tomography. DSCT scanners are equipped with two tubes and detectors at 90° to each other in a single gantry rotating at 330 ms. Additionally, in-vitro studies have shown that new detector technologies such as flat-panel CT scanners can provide significantly greater spatial resolution than traditional CT systems.16 This technology is expected to provide greater robustness for imaging of the coronary arteries, especially in patients with a high heart rate.17
Coronary Calcium Determination
Essential Point
Examination Techniques
Coronary Calcium Determination by Electron-Beam Computed Tomography
Agatston Calcium Score
Essential Point
Volume Calcium Score
Calcium Mass Determination
Essential Point
Coronary Calcium Determination by Multislice CT
Essential Point
Interpretation
Essential Point
Scanning Protocol
Reporting of Coronary Calcium Findings
Outlook
CT Coronary Angiography
Principle of Computed Tomography
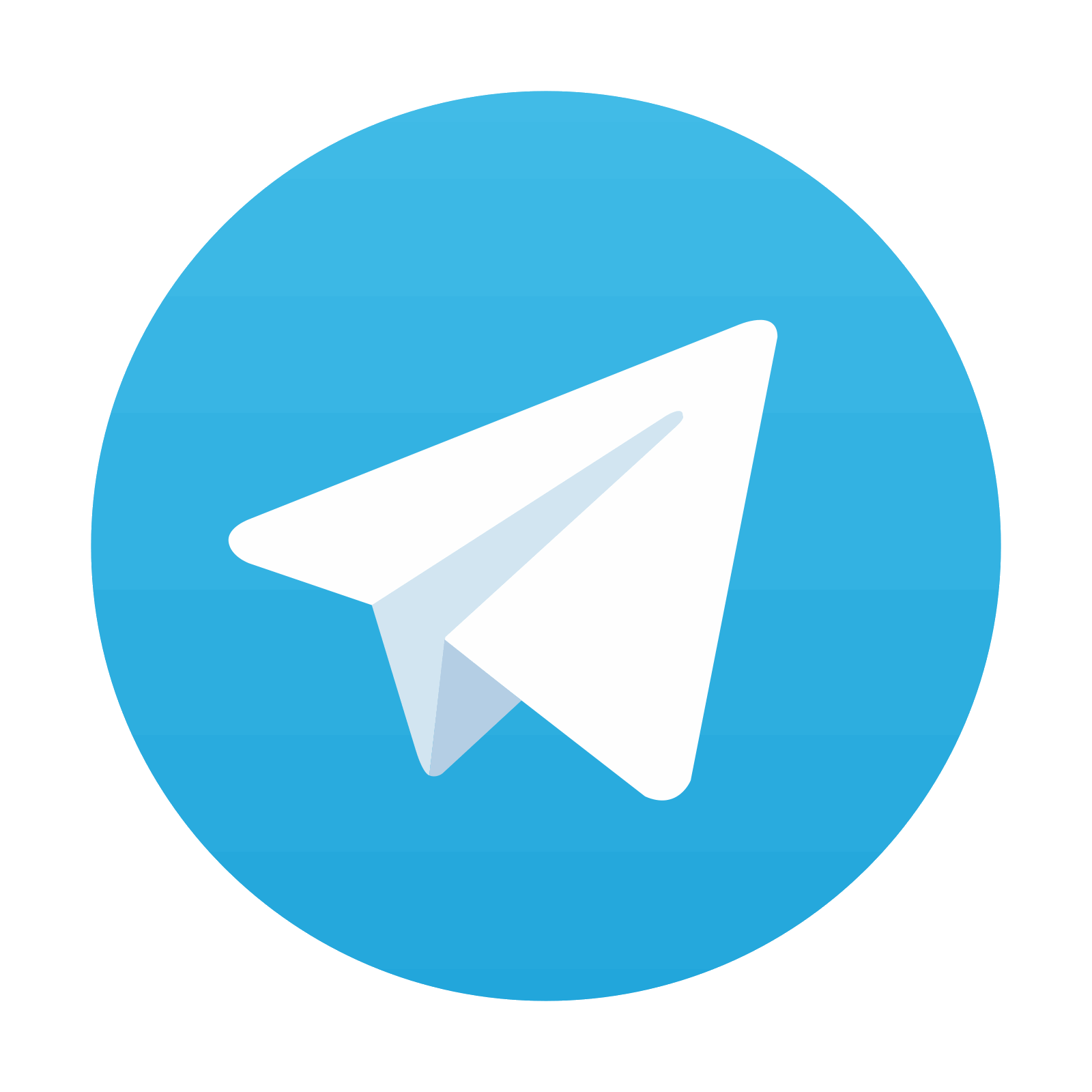
Stay updated, free articles. Join our Telegram channel
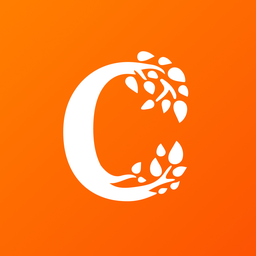
Full access? Get Clinical Tree
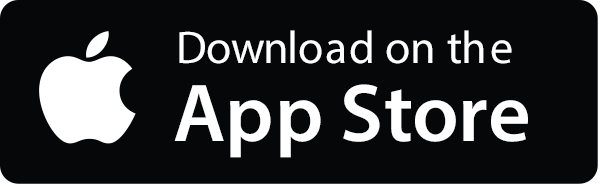
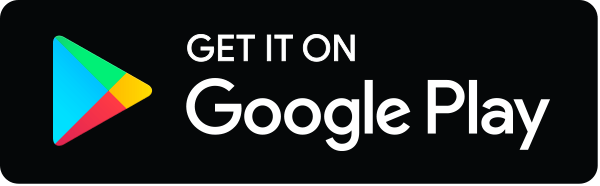