div class=”ChapterContextInformation”>
13. Advanced Coronary Artery Vessel Wall Imaging and Future Directions
Keywords
Coronary artery diseaseHigh-risk plaqueMagnetic resonance imagingComputed tomographyPositron emission tomographyCoronary artery disease (CAD) remains a menacing health threat in the industrialized countries despite recent decline in mortality. The majority of myocardial infarctions or sudden coronary deaths are caused by coronary plaque disruption and subsequent thrombotic occlusion, a process occurs suddenly after years of indolent atherosclerosis progression. Angiographic techniques that focus primarily on lumen narrowing have proven inadequate in accurately assessing lesion-specific risk as the majority of culprit plaques do not manifest significant stenosis. The composition and surface conditions of atherosclerotic plaques vary during different disease stages. Several types of lesion morphology, such as thin-cap fibroatheroma, are associated with weakened structure and predisposition for rupture. Plaques that are prone to rupture are referred to as “vulnerable” or “high risk.” Imaging techniques that are capable of directly detecting high-risk plaque features are highly desirable as they could potentially improve lesion-specific risk stratification and help guide therapeutic decision-making.
To address the unmet clinical needs for more precise lesion characterization, coronary vessel wall imaging is an active research area that is currently undergoing continuous development. Existing techniques are being improved to achieve better spatial resolution and diagnostic accuracy. Imaging time and complexity are being reduced and robustness being improved. New biomarkers and contrast mechanisms are being explored and validated. In this chapter we will briefly review the recent advances in noninvasive coronary vessel wall imaging techniques and discuss the trend toward comprehensive imaging solutions that consist of high-risk morphology, plaque burden, and pathophysiological activities. Additional discussions on coronary vessel wall imaging may also be found in the recent review articles on this topic [1–5].
New Advances in Coronary Vessel Wall MRI
Positive Remodeling and Plaque Burden
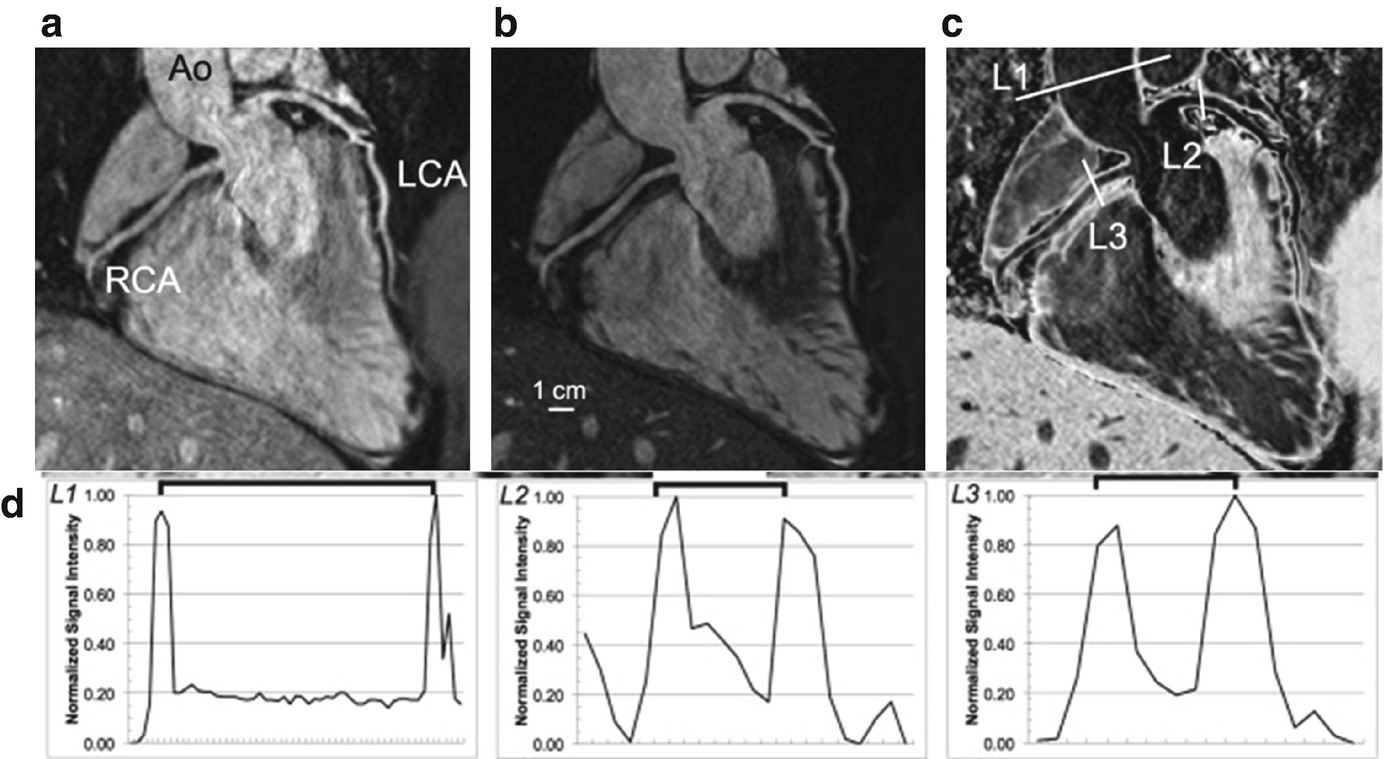
Reformatted images of a whole-heart vessel wall imaging using the i-T2prep acquisition. (a, b) The T2prep(−) and T2prep(+) acquisitions. (c) The aortic, LCA, and RCA vessel wall in weighted subtracted images. (d) The normalized pixel intensity of a profile across the vessel at the level of ascending aorta, LCA, and RCA. Ao ascending aorta, LCA left coronary artery, and RCA right coronary artery. (Reproduced from Andia et al. with permission [15])
Intra-plaque Hemorrhage and T1 Hyperintensity
Intra-plaque hemorrhage (IPH) is a known high-risk plaque feature that is associated with plaque progression and a predictor for future major adverse cardiovascular events [18, 19]. Existing data from autopsy studies and animal experiments suggest that IPH plays an important role in leukocyte infiltration and the enlargement of lipid core [18, 20]. Methemoglobin is the intermediate product in the process of hemoglobin breakdown which is present in the acute to subacute phases of IPH. It is a strong paramagnetic substance that markedly shortens T1; therefore, it can be visualized as regions of hyperintensity on T1-weighted black-blood techniques [21]. This technique has been well developed and validated to detect IPH in larger arteries such the carotid arteries [22]. Kawasaki et al. performed a comprehensive study comparing high-intensity plaques (HIPs) on T1-weighted black-blood images with plaque morphology on CT and intravascular ultrasound [23]. Increased frequency of adverse plaque characteristics was observed in HIPs, including higher positive remodeling, ultrasound attenuation, and spotty calcium. In another study comparing HIPs on MRI with CT plaque findings, Oei et al. observed significantly lower CT density in HIPs, suggesting a probable association between HIPs and IPH [24]. Notably, there was no correlation of plaque signal intensity with the degree of stenosis. The prognostic value of T1-weighted coronary MRI has been investigated by Noguchi et al. in a longitudinal study that followed 568 patients with suspected or known CAD for a median of 55 months [25]. Using plaque to myocardium signal ratio (PMR), the researchers found that patients with HIPs (PMR >1.4) experienced significantly higher rate of coronary events. The calculation of PMR is a simplified way to normalize plaque signal intensity and reduce the impact from coil sensitivity variations. The presence of HIP was determined as an independent predictor with a hazard ratio of 3.56. The same group recently applied the technique to 48 patients before and after 12 months of intensive statin therapy and observed significant decrease in PMR from 1.38 to 1.11, indicating a potential role of the technique for quantitative assessment of therapeutic effects [26].
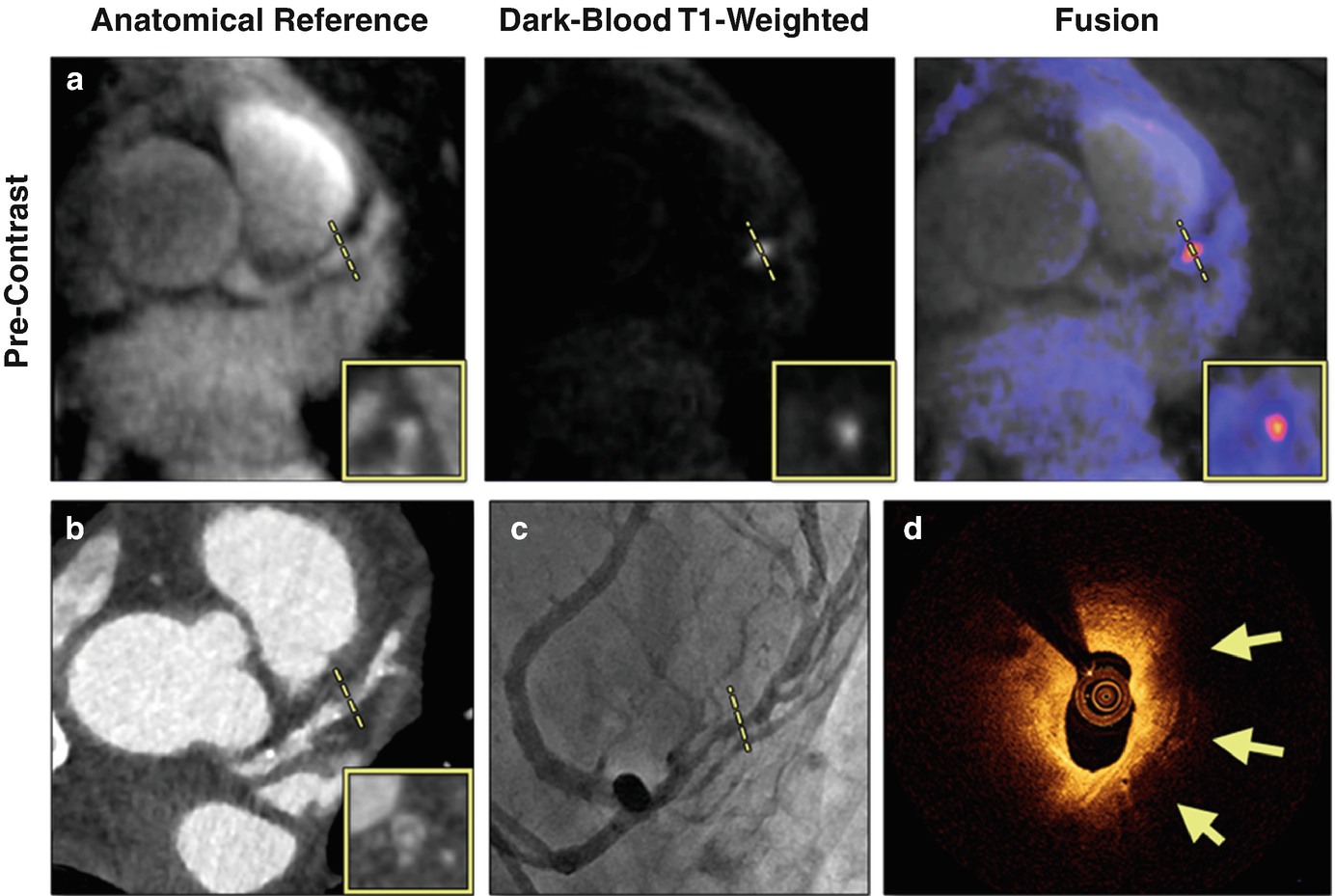
A case of suspected CAD patient with a HIP on non-contrast CATCH images. (a) Non-contrast T1-weighted, anatomical reference and fusion images. (b) Computed tomography angiography. (c) X-ray angiography. (d) Optical coherence tomography cross-sectional image at the corresponding location of the coronary hyperintensive plaque. Arrows point to signal-poor regions, suggesting a large lipid pool. Dotted lines represent the location and orientation of the cross-sectional images at the lesion which are shown in boxes. LAD left anterior descending. (Reproduced from Xie et al. with permission [27])
Although there is strong clinical evidence supporting the prognostic value of T1-weighted coronary imaging, the pathophysiological nature of plaque hyperintensity remains an active topic for research. Early study by Jansen et al. demonstrated in a small group of patients with acute coronary syndrome (N = 18) that coronary hyperintensity could result from intracoronary thrombus, which contains T1-shortening methemoglobin similar to IPH [28]. Ehara et al. also demonstrated the association between T1 hyperintensity and thrombus using intracoronary OCT as the reference [29]. Matsumoto et al. further investigated the relationship between the localization of T1 hyperintensity, such as intrawall or intralumenal, and the type of plaque morphology on OCT. Intrawall HIPs were associated with macrophage accumulation and absence of calcification, whereas intralumenal HIPs were associated with thrombus and intimal vasculature [30]. Xie et al. demonstrated that PMR is positively associated with the amount of high-risk plaque features including lipid core, macrophages, and cholesterol crystals as observed on OCT [27]. This result was later independently supported by a larger study of 106 patients with angina pectoris from which the investigators observed a stepwise increase in PMR of the culprit lesions in proportion to the accumulation of the number of adverse plaque characteristics [31]. In vivo validation of T1-weighted imaging for detecting coronary IPH is difficult due to the lack of gold standard for IPH. A recent study applied T1-weighted imaging to ex vivo coronary and carotid plaque specimens collected during endarterectomy surgery. Compared with histological analysis, T1-weighted imaging demonstrated sensitivity and specificity of 95.0% and 92.1%, respectively, for detecting IPH [32].
Contrast Enhancement of Vessel Wall
Gadolinium-based contrast-enhanced MRI (CE-MRI) is routinely used to boost the blood signal and improve the visibility of lumen for MR angiography. Alternatively, recent studies also demonstrated its capability to evaluate tissue properties of vessel wall and plaques, such as inflammation, neovascularization, and fibrosis. Compared with angiographic applications for which imaging is usually performed during arterial phase, vessel wall imaging typically requires sufficient delay after injection, e.g., 1 hour, to allow renal filtration and the washout of contrast media from healthy tissue. In one of the earliest attempts of CE-MRI for coronary vessel wall imaging, Maintz et al. studied nine patients with coronary artery disease confirmed by X-ray angiography. Using multidetector CT angiography as the reference, the investigators found that the majority of coronary plaques enhanced on CE-MRI (11 out of 13) were mixed plaques with partial calcification [21]. Further investigation using CT angiography and invasive coronary angiography as reference showed that coronary vessel enhancement is more prevalent in lesions that were calcified and with higher degree of lumen stenosis [33].
Contrast uptake in vessel wall is generally considered as the result of increased blood supply to the vascular bed as well as increased permeability of endothelium, both of which are associated with active inflammation. On the other hand, the “delayed enhancement” appearance is attributed to increased extracellular space with reduced washout kinetics, which are associated with fibrosis commonly found in recurrent or chronic inflammation [34]. Varma et al. investigated the feasibility of visual and quantitative assessment of vessel wall CE-MRI of atherosclerotic plaques in CAD and subclinical coronary vasculitis in systematic lupus erythematosus (SLE). The study showed that coronary vessel wall enhancement was prevalent in both diseases compared with controls that showed little signs of vessel wall uptake. Notably, the enhancement pattern appeared different for the two groups, with CAD patients showing patchy enhancement concentrated to the areas of minimal lumen diameter whereas SLE patients showing more diffused enhancement. Extensive coronary vessel wall enhancement was also observed in patients with Takayasu arteritis which is a type of chronic inflammatory disease of the aorta and its branches [35].
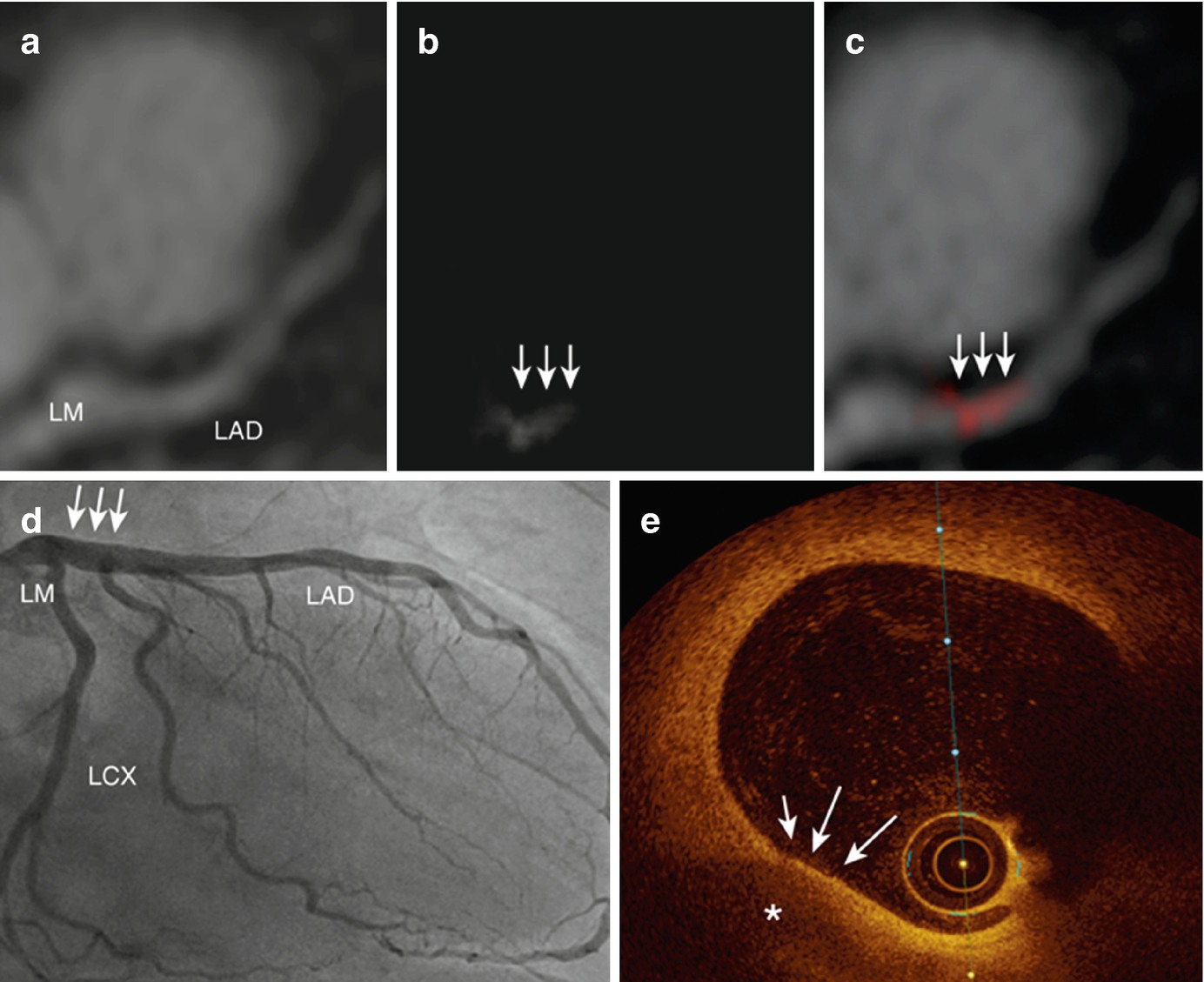
A case of gadofosveset-enhanced MRI of a 63-year-old patient with chest pain. (a) MR angiography shows the course of the LM and LAD. (b) Gadofosveset-enhanced MRI demonstrates contrast enhancement in the left main artery (white arrows). (c) Fusion of MR angiography and vessel wall imaging sequence. (d) X-ray coronary angiography shows no signs of significant luminal narrowing in the entire coronary artery tree. (e) OCT reveals a thin-cap fibroatheroma (white arrows) with a large lipid core (asterisk) in the proximal LAD, correlating with the location of increased signal enhancement on gadofosveset-enhanced MRI. LM left main, LAD left anterior descending. (Reproduced from Engel et al. with permission [41])
Vasomotion and Endothelial Functions
Vasomotion is an important physiological function of the arteries that is often impaired by atherosclerosis. Stress imaging of coronary artery vasomotion is performed commonly in the catheterization laboratory. MRI-based vasomotor evaluation is an emerging alternative that can be performed noninvasively. The pioneering work by Pepe et al. successfully demonstrated the use of 2D spoiled gradient-echo sequence for imaging the cross-sectional view of coronary arteries before and after pharmacological vasodilation induced by sublingual nitroglycerin [42]. Terashima et al. showed the feasibility of a similar protocol in 12 patients who also underwent X-ray angiography, with the MRI measurements showing low interobserver variability (<5%) and good correlation with X-ray angiography (r = 0.98) [43]. Hays et al. replaced the pharmacological stressor with the use of isometric handgrip, which allowed the evaluation of endothelial-dependent vasomotor response [44]. The study on 20 healthy subjects and 17 patients with CAD demonstrated that coronary artery area increased in healthy adults with stress but decreased in CAD patients. MRI has also been used to quantify coronary vessel wall distensibility, which is defined as the ratio of lumen diameter or area between diastole and systole [45]. This technique was applied to a group of heart transplant recipients and showed that coronary distensibility was correlated with wall thickness [46].
In summary, recent developments in MRI-based coronary vessel wall imaging have demonstrated its feasibility for evaluating various important disease characteristics including morphology, tissue properties, and functions. The existing protocols typically requires ECG gating and/or respiratory navigator. Due to the limitations in motion sensitivity, spatial resolution, coverage, and imaging time, data quality is often not sufficient in a considerable proportion of patients. Recent developments in motion compensation and fast imaging techniques showed promises to improve upon or eliminate these shortcomings, but additional clinical experience and data are needed to sufficiently validate the claims. Further technical improvements are still required to allow applications in patients with more challenging imaging conditions such as arrhythmia or the presence of stents or metal implants. Last but not least, it is important to improve the portability and availability of the new techniques and standardize study protocols in order to facilitate the clinical translation.
New Advances in Coronary Vessel Wall CTA
Technological Advances in CT
In recent years, there have been significant advances in CT hardware and software, which have expanded the clinical utility of CT for cardiovascular imaging. Imaging of the coronary arteries is challenging due to their small dimensions, tortuosity, and continuous motion. Cardiovascular CT needs full synchronization with the electrocardiogram (ECG) signal and high temporal resolution to “freeze” cardiac motion. A general technical improvement for CT scanners is that gantry rotation times have been decreased by the manufacturers to address the requirement of high temporal resolution for clinical cardiac CT. Concurrently, there has been an effort to increase the spatial resolution, as well as the z-coverage to facilitate faster scanning of the heart. For low (<60 beats/min) and regular heart rates, single-heartbeat scans can be performed. Major advances in current CT hardware have been highlighted in a recent review article [47].
Coronary Calcium Scoring
Coronary calcium scoring with non-contrast CT is used worldwide for cardiovascular risk stratification and provides a measure of global coronary atherosclerotic burden. It is a simple and low-cost test, without the use of premedication or contrast, and with a uniformly low attendant radiation burden [48–50]. To date, several follow-up studies have reported that the total coronary artery calcium measured by non-contrast CT predicts cardiovascular events [51–65], beyond standard cardiovascular risk indices.
Coronary CT Angiography (CTA)
Coronary CT angiography (CTA) has recently emerged as a noninvasive diagnostic test in selected stable but symptomatic patients with suspected CAD [66–71]. Current clinical interpretation of CTA relies on visual assessment of stenosis grade, plaque type, and presence of high-risk plaque features [72, 73]. Invasive coronary angiography is the gold standard for the diagnosis of obstructive stenosis. CTA has, to date, shown high accuracy and sensitivity for detecting coronary artery stenoses when compared to invasive coronary angiography [67–71]. In particular, CTA has shown very high negative predictive value for obstructive stenoses (range 89–99% in multicenter studies) in patients with symptoms that suggest the presence of coronary artery disease [67–71].
In the last decade, numerous large-scale randomized, controlled trials have established the efficacy of CTA as a diagnostic test in clinical care. In the prospective, randomized PROMISE trial with 10,003 patients, performance of CTA was equivalent to stress testing when used as an initial test for suspected CAD, with similar event rates between stress testing and the CTA group at 2-year follow-up (3.3% vs. 3.0%) [74]. In the prospective, randomized SCOT-HEART trial, 4146 patients with suspected CAD were randomly assigned to standard care alone (typically, stress testing) or standard care plus CTA [75]; in this trial, CTA was shown to clarify the diagnosis, enable appropriate treatment, and significantly improve patient outcomes [76].
Plaque Imaging
Current clinical interpretation of CTA relies on subjective visual assessment of stenosis grade or the detection of obstructive disease (≥50% stenosis). Beyond stenosis, CTA also permits noninvasive assessment of atherosclerotic plaque (including plaque burden and composition) and outward coronary artery remodeling [77–81]. Studies have shown that coronary plaque volume and remodeling quantified from CTA correlate strongly with invasive intravascular ultrasound (IVUS) [79, 82–86]. In a blinded comparison between CTA and IVUS by Dey et al., NCP volumes measured using standardized semiautomated software showed excellent per-plaque correlation (r = 0.94), with no significant differences compared to IVUS measurements [84].
Coronary CTA Plaque Features for Prediction of Adverse Cardiovascular Outcomes
Features of plaque vulnerability measured by CT have been reported to include low-attenuation or low-density plaque (LAP)—with attenuation values ≤30 Hounsfield units (HU)—corresponding to the necrotic core and positive or outward remodeling [81, 87–90]. The napkin-ring sign has also been shown to be a CT signature of high-risk coronary atherosclerotic plaque [89, 91, 92].
Kristensen et al. measured CT plaque volumes with a semiautomated method in a study of 312 patients who presented with non-ST-segment elevation myocardial infarction at baseline. They showed that major adverse cardiac events (MACE) were associated with a higher amount of noncalcified plaque (NCP) in nonobstructive coronary lesions [93]. Semiautomated plaque measurements have been shown to improve prediction of future acute coronary syndrome (ACS) over conventional CTA assessment [receiver operator characteristic area under curve (AUC) 0.79 vs. 0.64, p < 0.05] [94].
A study by Motoyama et al. investigated whether visually assessed plaque characteristics by CTA can predict midterm likelihood of future ACS over mean 3.9 years of follow-up [95]. The presence of LAP, positive remodeling, and severe stenosis by CTA (≥70%)—defined as high-risk plaque features—were visually assessed in 3158 patients undergoing CTA. ACS occurred in 88 patients and was significantly more frequent in patients with severe stenosis; CTA-verified high-risk plaque was an independent predictor of ACS. However, the cumulative number of patients suffering ACS was similar for patients with and without visually identified high-risk plaques [95]; this was primarily attributed to the diffuse nature of atherosclerosis.
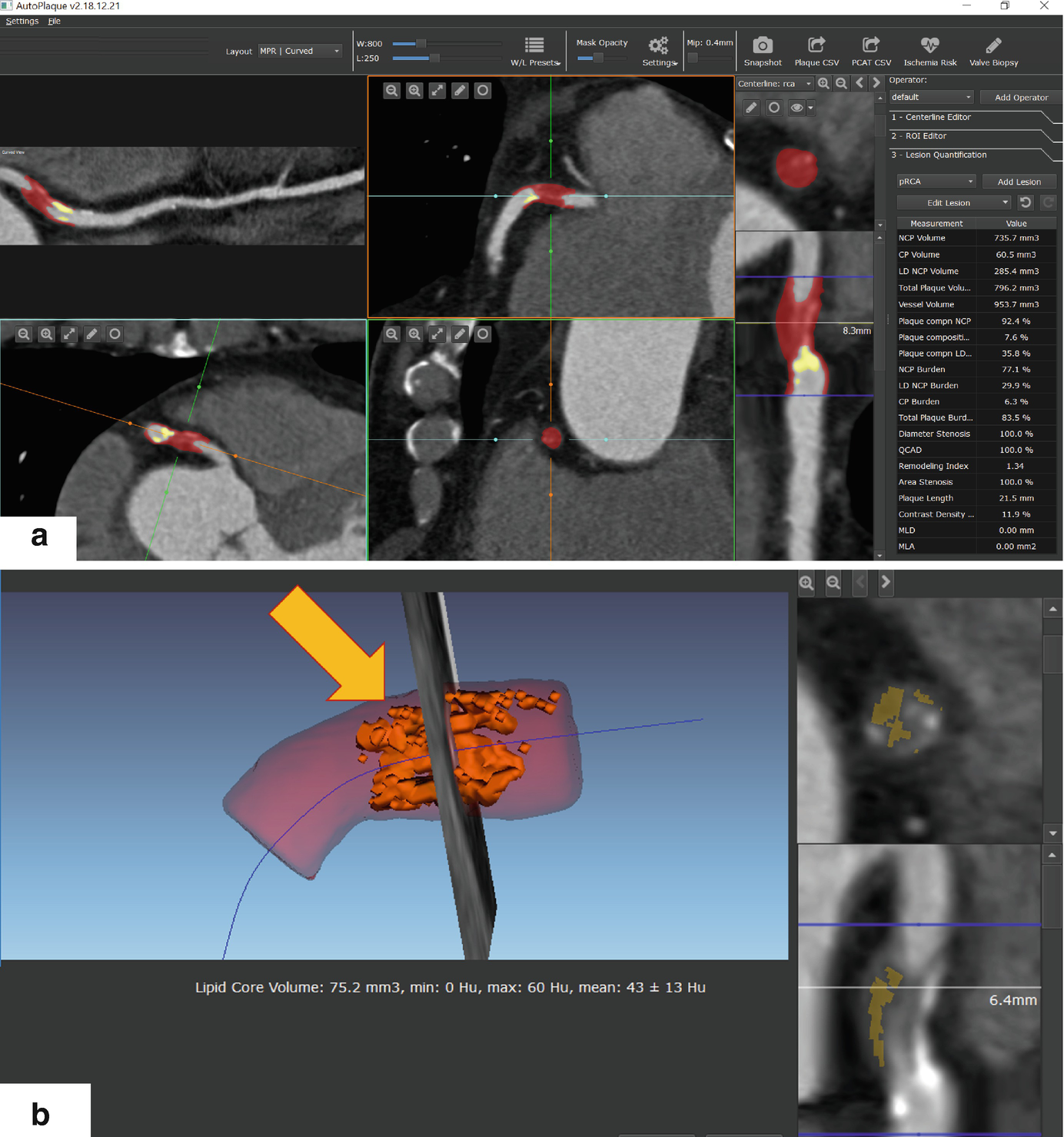
Example of semiautomated quantification of high-risk coronary plaque with large NCP burden, lipid core, and coronary artery remodeling, in a 66-year-old male patient undergoing CTA. (a) Standardized quantification of plaque and stenosis, red overlay shows noncalcified plaque, and yellow overlay shows calcified plaque. (b) Detailed view showing lipid core or low-density noncalcified plaque. Arrow shows 3D view of lipid core, shown in yellow overlay in cross-sectional view
Plaque Progression for Prediction of Adverse Cardiovascular Outcomes
Intravascular imaging studies have demonstrated that the lesions likely to result in MACE have modest luminal stenosis and large plaque burden but evolve voluminously to result in significant stenosis at the time of ACS [7, 98, 99]. The prognostic importance of plaque progression has also been highlighted in the CTA study by Motoyama et al. [95]. Serial CTA was performed in 449 patients, and plaque progression was visually assessed as any increase in stenosis grade [95]; plaque progression detected by serial CTA was found to be an independent predictor of ACS.
New data using standardized quantitative software show that a per-patient low-density lipoprotein (LDL) cholesterol decrease of 10% or greater is related to beneficial changes in the amount and composition of noncalcified plaque by serial CTA performed 1 year or more apart [100]. Recently, it has also been shown in a multicenter registry that statin therapy causes slower progression of total plaque volume, along with increased calcification and reduction of high-risk plaque features by CTA [101].
Plaque and Lesion-Specific Ischemia
It is known that stenosis does not equate ischemia, and significant stenosis in CTA often does not indicate hemodynamic significance. Low-density NCP—which has been shown to be of prognostic importance—has also been shown to be an imaging biomarker which predicts lesion-specific ischemia by invasive FFR [102, 103]. In the multicenter NXT trial, low-density NCP provided independent and incremental discrimination of ischemia beyond stenosis severity [102].
Plaque and Machine Learning
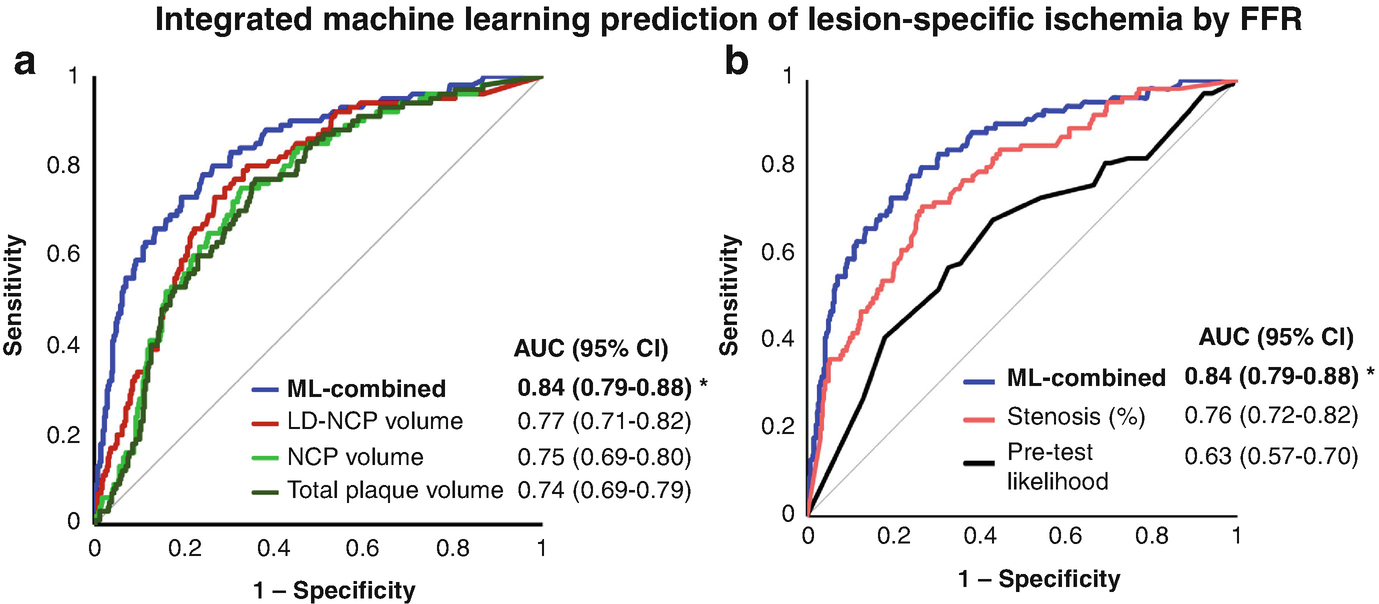
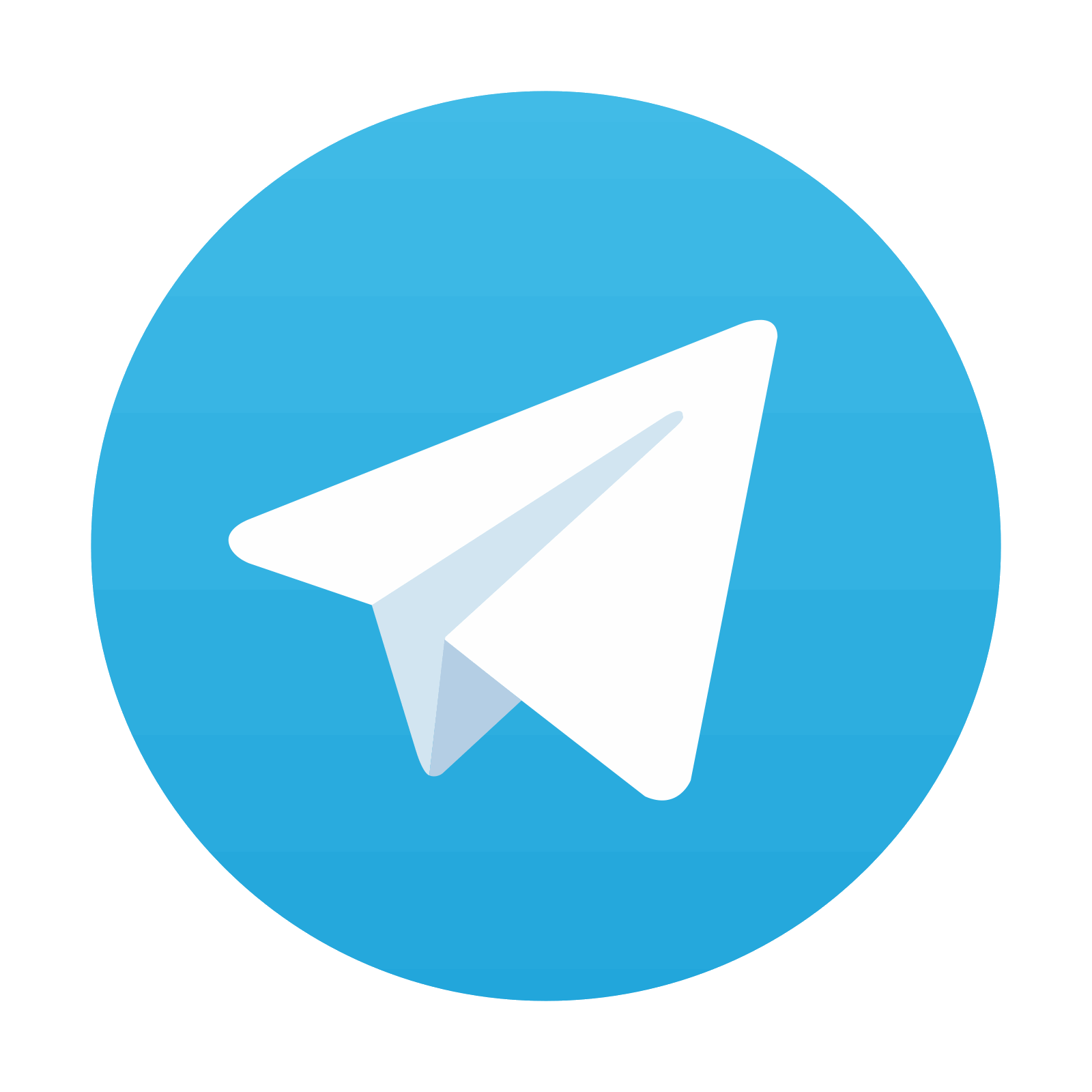
Stay updated, free articles. Join our Telegram channel
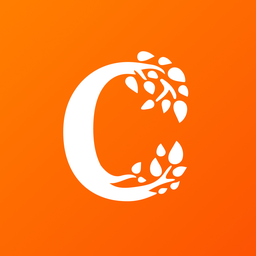
Full access? Get Clinical Tree
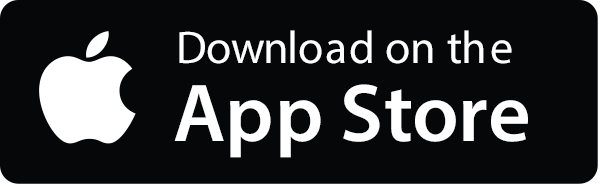
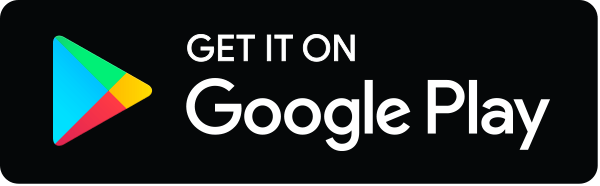