Target structures
Scan mode
Respiration status
Extracardiac structures only
Non-ECG-synchronized spiral
Free breathing or breath holding
Intracardiac structures and coronary arteries
Prospectively ECG-triggered sequential
Free breathing (with or without respiratory triggering)
Retrospectively ECG-gated spiral
Breath holding, controlled ventilation with or without general anesthesia, or free breathing (young children)
Dynamic evaluation of airways
Four-dimensional sequential (supplementary)
Free breathing
Regional lung perfusion (iodine) and ventilation (xenon)
Dual-energy spiral
Free breathing or breath holding
Conventional non-ECG-synchronized spiral scanning has been used to evaluate extracardiac vascular and airway abnormalities of pediatric congenital heart disease [1–8]. Recently introduced high-pitch (up to 3.0–3.4; table feed, 41.1–46.6 cm/s) dual-source spiral scanning allows considerable reduction of cardiac and respiratory motion artifacts on non-ECG-synchronized cardiac CT [9, 10]. Nonetheless, ECG-synchronized scanning with either retrospective ECG gating or prospective ECG triggering should be performed for the evaluation of intracardiac structures and coronary arteries (Fig. 18.1). This has been proven by improved coronary artery visibility on ECG-synchronized cardiac CT in children with congenital heart disease [11–15]. ECG-synchronized cardiac CT also allows more accurate identification and more detailed characterization of intracardiac abnormalities, e.g., potentially life-threatening coronary sinus ostial atresia with a persistent left superior vena cava (SVC) that has not been detected on cardiac CT [16].
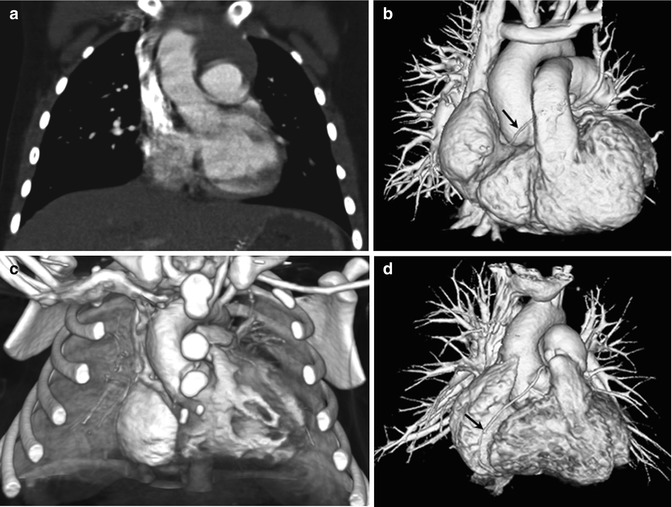
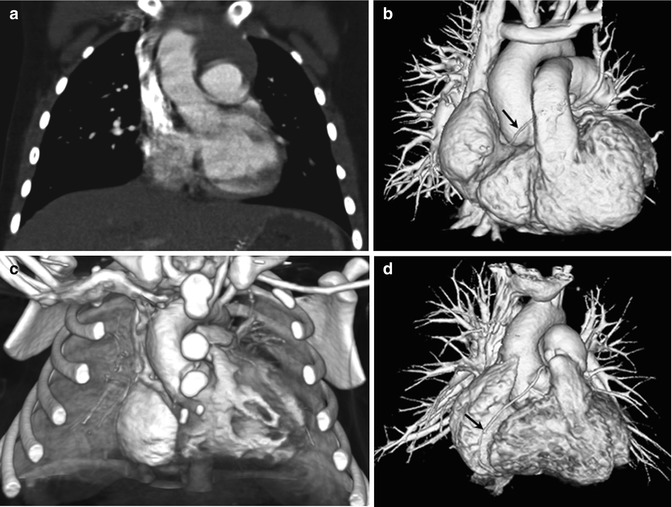
Fig. 18.1
CT scan techniques for pediatric congenital heart disease. (a) Coronal CT image acquired with breath-hold single-source non-ECG-synchronized spiral scanning in a 5-year-old girl shows cardiac pulsation artifacts. (b) Volume-rendered CT image acquired with free-breathing dual-source high-pitch non-ECG-synchronized spiral scanning in a 6-year-old girl reveals no discernible cardiac and respiratory motion artifacts. The right coronary artery (arrow) is faintly seen. (c) Volume-rendered CT image acquired with free-breathing dual-source prospectively ECG-triggered sequential scanning in a 2-day-old male newborn shows excellent image quality without cardiac and respiratory motion artifacts. (d) Volume-rendered CT image acquired with breath-hold dual-source retrospectively ECG-gated spiral scanning shows excellent image quality without cardiac and respiratory motion artifacts. The right coronary artery is clearly shown (arrow)
The best cardiac phase with minimal cardiac motion should be selected for ECG-synchronized CT scanning [5, 6]. The mid-diastolic phase, the so-called diastasis, is chosen at low heart rates, while the end-systolic phase generally offers the best image quality at high heart rates (i.e., >75 beats/min). This heart rate-dependent best cardiac phase is attributed to the fact that the diastasis is decreased and eventually disappeared with increasing heart rate, and the end-systolic cardiac rest period tends to remain constant irrespective of heart rate. Single cardiac phase is usually sufficient for the morphologic evaluation, while multiphase scanning may be necessary for the accurate assessment of ventricular function, ventricular volumes, and coronary arteries at the expense of higher radiation dose.
High temporal resolution is beneficial for ECG-synchronized pediatric cardiac CT because most children have high heart rates. Currently, there are technical limitations to improve temporal resolution by increasing gantry rotation speed (0.27–0.35 s for single-source CT, 0.28–0.33 s for dual-source CT) and pitch. High gravitational (g) forces associated with fast gantry rotation speed (e.g., 17 g for 0.42 s rotation time, 33 g for 0.33 s rotation time) are close to a current mechanical upper limit. It is attributed to the fact that the g force is inversely proportional to the square of gantry rotation time. Theoretically, there are certain limits in increasing pitch in order to obtain gapless image data. In this respect, heart rate-dependent pitch, if available, is more beneficial than fixed low pitch. The highest temporal resolution, 75–83 ms, of ECG-synchronized scan is currently achieved with dual-source CT because only a quarter of gantry rotation is required for image reconstruction [9]. In contrast, at least a half of gantry rotation is needed for image reconstruction of ECG-synchronized scan using a single-source CT system.
Cine CT has been used to evaluate tracheobronchomalacia [17], air trapping [18], and subtle lung parenchymal abnormalities simply by reducing motion artifacts [19] in free-breathing children. With a longer longitudinal coverage of modern multi-detector row CT (MDCT) (4–16 cm for 64–320 detector rows), four-dimensional (4D) airway CT is increasingly used in clinical practice [5, 6, 20]. This 4D airway CT can overcome diagnostic pitfalls of conventional cine CT resulting from longitudinal motion of the airways and oblique course of the bronchi. In general, expiratory airway collapse greater than 50 % has been regarded as a diagnostic criterion of tracheobronchomalacia in children. A new diagnostic criterion, e.g., expiratory tracheal collapse of approximately 31–32 % in a study [17], and a quantitative analysis method should be developed for free-breathing children (Fig. 18.2).
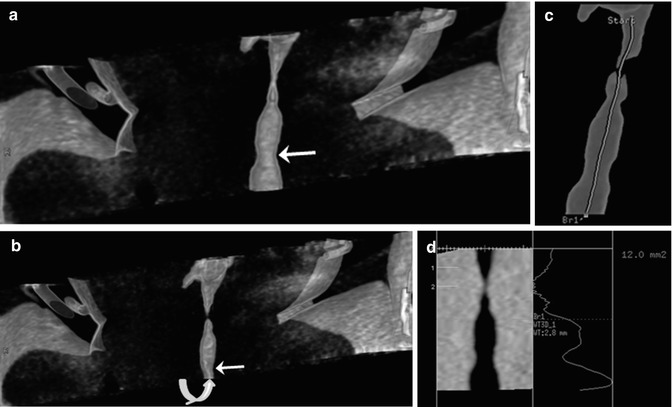
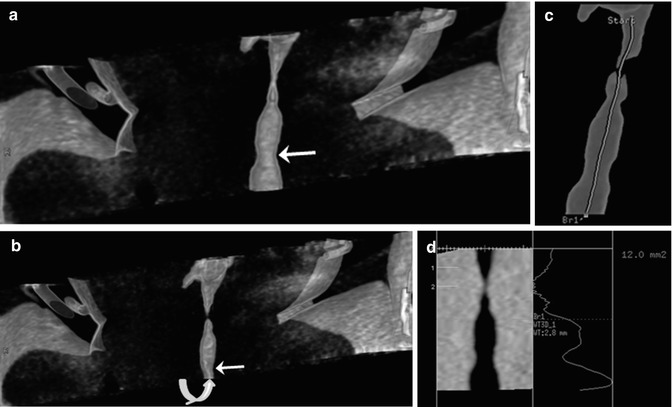
Fig. 18.2
Four-dimensional airway CT. Inspiratory (a) and expiratory (b) coronal volume-rendered CT images demonstrate a mild subglottic stenosis (arrow) and tracheomalacia (curved arrow). (c) The airway central axis (green line) is semiautomatically traced for quantitative analysis. (d) The cross-sectional areas of the airways were automatically measured along the airway central axis, which is mandatory for quantitative assessment of airway abnormalities, such as tracheobronchomalacia
Dual-energy CT is a recently highlighted scan mode acquired at two different tube voltages for material differentiation, e.g., iodine for perfusion and xenon for ventilation that is not readily seen on conventional single-energy CT [21]. Regional lung perfusion and ventilation can be assessed with dual-energy CT [22–27]. In children with congenital heart disease, dual-energy CT may be used for initial identification and follow-up of pulmonary embolism [22, 28] (Fig. 18.3) and for evaluation of airway anomalies or diseases. Radiation dose of dual-energy CT using a dual-source system is comparable to that of single-energy CT [21], whereas dual-energy CT using a single-source system with rapid tube voltage switching delivers substantially higher radiation dose [29]. The difference in radiation dose between the two dual-energy CT techniques should be cautiously considered in children.
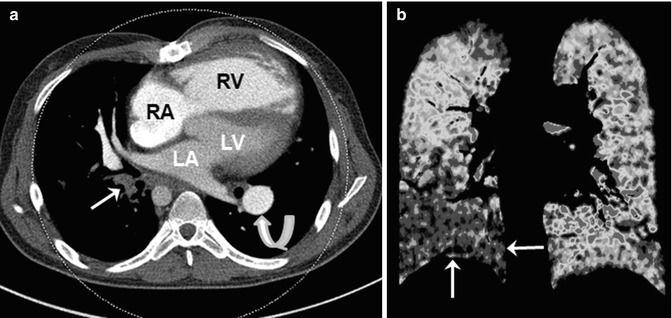
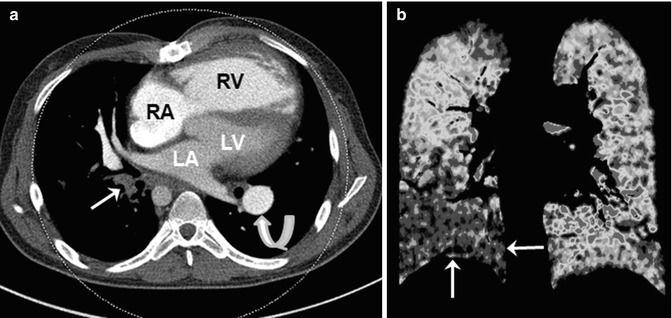
Fig. 18.3
Dual-energy CT for evaluating pulmonary embolism and lung perfusion. (a) Axial weighted average CT image in a 22-year-old man with repaired tetralogy of Fallot and recurrent pulmonary valve endocarditis shows obstructing pulmonary embolism (arrow) in the right lower lobe. The right ventricle (RV) appears enlarged and hypertrophic. A patent and dilated left pulmonary artery branch (curved arrow) is noted. (b) Coronal color-coded iodine map reveals a large perfusion defect in the right lower lobe (arrows). RA right atrium, LA left atrium, LV left ventricle
Radiation Dose Reduction Strategies
To pursue the principle of as low as reasonably achievable in children, radiation dose of cardiac CT should be optimally minimized without compromising diagnostic image quality. In fact, submillisievert cardiac CT examination can be achieved by using available dose reduction strategies collectively, including body size-adapted dose settings, attenuation-based tube current modulation, optimally low tube voltage, ECG-controlled tube current modulation, minimal longitudinal coverage, and an iterative reconstruction algorithm [5, 6, 13, 15, 30].
First of all, radiation dose settings should be adjusted to individual body size. Body weight, body mass index, cross-sectional dimensions, and mean body density can be used to develop a body size-adapted cardiac CT protocol [31–33]. In general, cross-sectional dimensions, such as body diameter and area, show better dose adaptation to body habitus than traditionally used body weight and body mass index do.
Tube current modulation should be used to reduce radiation dose of cardiac CT without degrading image quality [5, 6, 30–34]. It may be classified into angular, z-axis, and combined types according to modulation direction. For its proper use, we first need to understand that basic principles differ between vendors [35]. In a study [34], radiation dose of pediatric cardiac CT could be reduced by 9 ~ 26 % by using combined tube current modulation. The degree of dose reduction is influenced by tube voltage and scan direction [36]. More importantly, we must ensure patient positioning in the gantry isocenter to avoid adverse effects of improper positioning. We also should clearly recognize that tube current modulation is not truly automatic and it needs user-defined target image quality.
Low tube voltage provides not only potential for radiation dose reduction but also higher contrast-to-noise ratio of cardiac CT [30, 37]. Depending on body size and scan technique, 80 or 100 kV has been used for pediatric cardiac CT to have the benefits of low tube voltage. Additionally, 70 kV recently became available for pediatric cardiac CT. However, it should be noted that tube current saturation is more frequently reached during tube current modulation at low tube voltage, which limits the dose-reducing effect of tube current modulation [6, 30]. The use of lower pitch is a practical solution to this problem. In this respect, we have to ensure that low tube voltage for cardiac CT is truly dose efficient and optimal.
ECG-controlled tube current modulation or ECG pulsing is a useful dose-saving technique for retrospectively ECG-gated spiral CT scanning, in which the full dose is applied to the target cardiac phase and 4 or 20 % of the full dose is applied to the rest of the cardiac cycle [5, 6, 38]. Heart rate-adapted pitch may further reduce radiation dose with increasing heart rate [39]. In contrast, prospectively ECG-triggered sequential scanning is an intrinsically low-dose imaging technique [5, 6, 15].
Although required scan range of cardiac CT is quite variable according to clinical requests, it should be kept to the minimum. Overranging, i.e., unnecessary radiation exposure outside a scan range of multi-slice spiral CT, is directly proportional to beam collimation, reconstructed slice thickness, and pitch [40]. Because this overranging has a great impact on total radiation dose in pediatric cardiac CT, it should be reduced by using the adaptive collimation technology [41]. However, this adaptive collimation technology is not available for high-pitch dual-source spiral CT scanning, resulting in a greater radiation exposure. Therefore, this high-pitch scanning should be used only when the favorable risk-benefit ratio is expected.
Recently introduced iterative reconstruction algorithms have potential to reduce radiation dose of pediatric cardiac CT, compared with conventional filtered back projection algorithms [42, 43]. Typical dose estimates of dose-optimized pediatric cardiac CT are below 1 mSv for single-source non-ECG-synchronized spiral scanning and prospectively ECG-triggered sequential scanning, 1–3 mSv for high-pitch dual-source spiral scanning, and 2–6 mSv for retrospectively ECG-gated spiral scanning in our institution. However, comprehensive single- or multicenter studies on radiation dose of pediatric cardiac CT are necessary to determine the current risk level of dose-optimized pediatric cardiac CT.
Clinical Applications
Recent technical innovations of cardiac CT expand clinical applications in patients with congenital heart disease. In addition to traditional applications including the evaluation of extracardiac great vessels, airways, and lungs, newer applications include the assessment of coronary arteries, conotruncal structures, intracardiac structures, cardiac function, ventricle volumetry, dynamic airway obstruction, and regional lung perfusion [44].
Aorta
Anomalies of the aorta commonly include obstructive lesions in the aortic arch, such as coarctation of the aorta (CoA), interrupted aortic arch (IAA), and aortic arch atresia [45, 46]. In CoA, CT can avoid diagnostic pitfalls of echocardiography resulting from geometric distortion caused by a large patent ductus arteriosus (PDA). Because a periductal aortic stenosis in CoA is a dynamic process, monitoring of blood pressure difference between upper and lower extremities and echocardiographic follow-up are necessary although a patient is initially asymptomatic without a definite evidence of CoA. If there are equivocal findings on echocardiography, cardiac CT can provide the accurate diagnosis of CoA (Fig. 18.4). The presence of collateral arteries indicates long-standing, severe CoA, and these collateral arteries can be seen on cardiac CT as early as 3 months of age (Fig. 18.4). In CoA, the affected aortic arch may show various degrees of hypoplasia and elongation that may lead to modified surgical techniques to develop optimal geometry of the aortic arch and avoid postoperative left main bronchus narrowing.
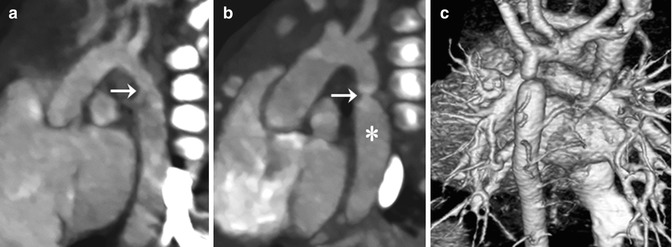
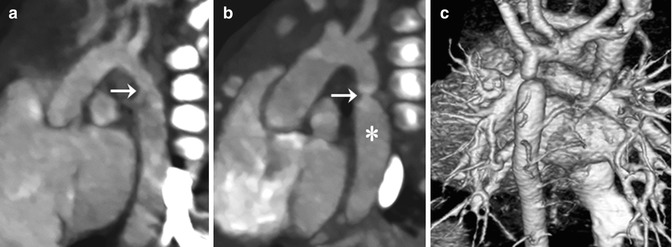
Fig. 18.4
Coarctation of the aorta. (a) CT image with an aortic arch view shows hypoplasia of distal arch and a minimal periductal stenosis (arrow). (b) Follow-up CT image demonstrates the development of a significant discrete narrowing (arrow) at the periductal area. The post-stenotic dilatation of the proximal descending thoracic aorta (asterisk) is noted. (c) Volume-rendered CT image with a posterior view reveals multiple collateral arteries connecting to the descending aorta, indicating significant aortic flow obstruction due to the coarctation of the aorta
Pseudocoarctation is characterized by tortuous elongation of a higher aortic arch without hemodynamically significant obstruction. In some cases showing pseudocoarctation on cardiac CT, cardiac catheterization may be necessary to exclude hemodynamically significant obstruction. Various degrees of progressive aneurysmal dilation of the aorta are shown in children with congenital connective tissue disorders, such as Marfan syndrome, Loeys-Dietz syndrome, and arterial tortuosity syndrome [47–49]. Cardiac CT can be used to detect and monitor cardiovascular complications in these connective tissue disorders (Fig. 18.5).
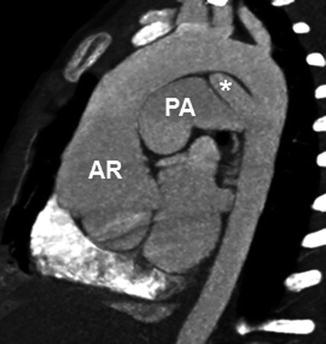
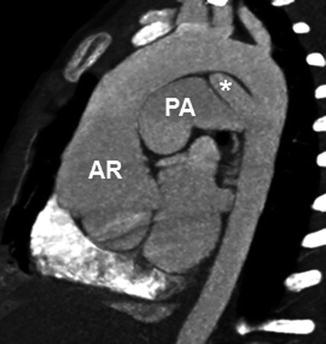
Fig. 18.5
A 2-year-old girl with Loeys-Dietz syndrome. CT image with an aortic arch view shows aortic root dilatation and annuloectasia. Other arteries, including the pulmonary arteries and aortic arch vessels, also show diffuse dilation. A patent ductus arteriosus (asterisk) is noted. AR aortic root, PA pulmonary artery
Among various types of aortic arch anomalies, common symptomatic vascular rings include double aortic arch and right aortic arch with an aberrant left subclavian artery (with or without Kommerell diverticulum) [50]. The arch anatomy and tracheal and esophageal compression can be accurately depicted on cardiac CT [51, 52]. The presence of the posterior ligamentum arteriosum contralateral to the aortic arch is closely related to the formation of vascular rings. However, the ligamentum arteriosum is not discernible on imaging. In this respect, the ligamentum arteriosum calcification, if detected, may be helpful to determine its location on cardiac CT [53]. Tracheomalacia may be complicated with these vascular rings before and more frequently after surgery [54–56]. Circumflex retroesophageal aorta makes surgical correction of aortic arch anomalies more difficult [57, 58] (Fig. 18.6).
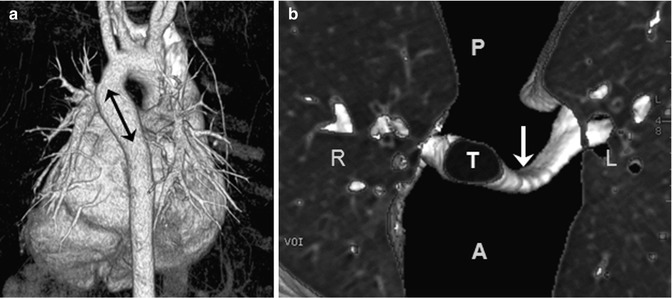
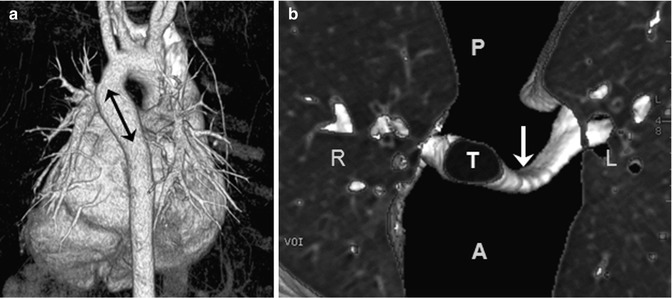
Fig. 18.6
Circumflex retroesophageal aorta. (a) Volume-rendered CT image with a posterior view shows the left aortic arch, a retroesophageal aortic segment (arrow), and the right-descending aorta. (b) Three-dimensional lung and airway CT image with a superior view reveals a left main bronchus narrowing (arrow) compressed by the aortic anomaly. T trachea, A anterior, P posterior, R right, L left
In addition to the aortic arch, aortic stenosis may occur at supravalvular and valvular levels. Supravalvular aortic stenosis, defined as a focal or diffuse narrowing of the aorta starting at the sinotubular junction, is commonly associated with Williams syndrome or may occur in nonsyndromic forms [59, 60]. Bicuspid aortic valve is seen in 3–8 % of patients with congenital heart disease and often associated with aortic stenosis and arteriopathy showing progressive dilation of the proximal aorta even in the absence of significant aortic stenosis [61]. In patients with aortic stenosis, cardiac CT may show anatomic features of valvular stenosis by planimetry, left ventricular hypertrophy, and dilation of the proximal aorta.
Pulmonary Artery
Obstructive pulmonary artery abnormalities, such as hypoplasia, stenosis, and atresia, can be shown on cardiac CT. These abnormalities are universally seen in patients with tetralogy of Fallot (TOF)/pulmonary atresia spectrum [62–64]. Juxtaductal left pulmonary artery stenosis often results in asymmetrically reduced left pulmonary vascularity on cardiac CT. Peripheral pulmonary artery stenosis is frequently seen in patients with Williams and Alagille syndromes [65, 66] (Fig. 18.7). In order to restrict pulmonary blood overflow resulting in pulmonary vascular disease, pulmonary artery banding is placed most commonly at the main pulmonary artery or at the branch pulmonary artery bilaterally as a hybrid procedure for hypoplastic left heart syndrome. Complications of this procedure, such as pulmonary artery stenosis resulting from impingement, distal migration, or scarring of the banding site and pulmonary artery pseudoaneurysm, can be identified on cardiac CT.
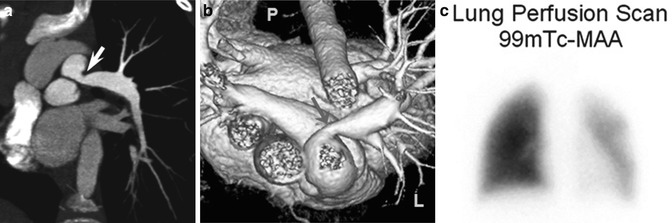
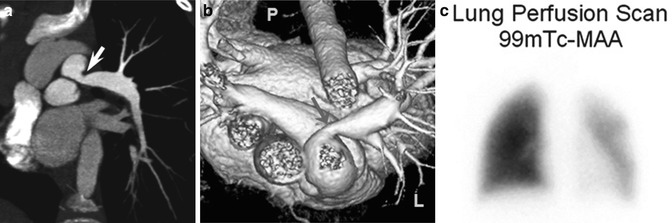
Fig. 18.7
A 7-year-old body with Alagille syndrome. Oblique coronal (a) and superior volume-rendered (b) CT views show a proximal left pulmonary artery stenosis (arrow). (c) Lung perfusion scintigraphy confirms diffusely decreased left-lung perfusion (25 % of total lung perfusion). P posterior, L left
In pulmonary atresia, the systemic arterial source of pulmonary circulation may be single or multifocal, including a PDA or major aortopulmonary collateral arteries (MAPCAs). Anatomic details of the central pulmonary arteries and various systemic arterial sources, crucial for surgical planning of unifocalization, can be delineated on cardiac CT [67] (Fig. 18.8). Thanks to such CT road map of MAPCAs, procedure time of catheter angiography and possibility of overlooked MAPCAs can be substantially reduced. Nonetheless, conventional catheter angiography is necessary for identifying communications between pulmonary arterial feeders.
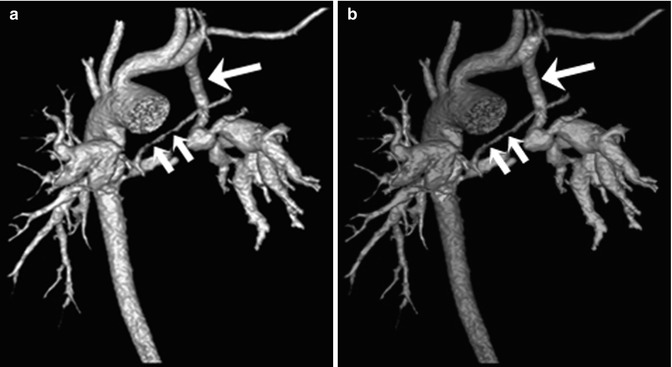
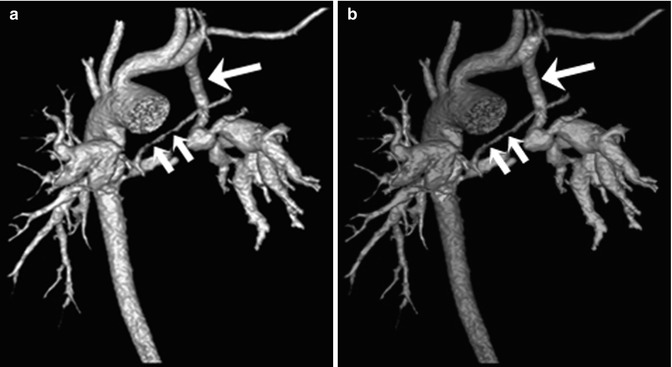
Fig. 18.8
Pulmonary atresia with major aortopulmonary collateral arteries (MAPCAs). (a) Volume-rendered CT image with an anterior view shows anatomic details of the tiny central pulmonary arteries (short arrows) and MAPCAs. A left modified Blalock-Taussig (LMBT) shunt (long arrow) is noted. (b) Color-coded volume-rendered image demonstrates three MAPCAs with different colors (green, light blue, and purple). The tiny central pulmonary arteries (short arrows) are connected to a green-colored MAPCA and a LMBT shunt (long arrow) is connected to a purple-colored MAPCA
Dilatation of the pulmonary arteries is commonly seen in patients with repaired TOF and absent pulmonary valve syndrome as a result of long-standing pulmonary regurgitation, or in patients with pulmonary arterial hypertension. In absent pulmonary valve syndrome, bronchial compression by severely enlarged central pulmonary arteries usually results in respiratory difficulties necessitating surgical treatments, such as reduction pulmonary arterioplasty [68, 69] (Fig. 18.9). Lung perfusion defects may be identified on dual-energy CT in patients with pulmonary arterial hypertension and pulmonary embolism [22, 70, 71].
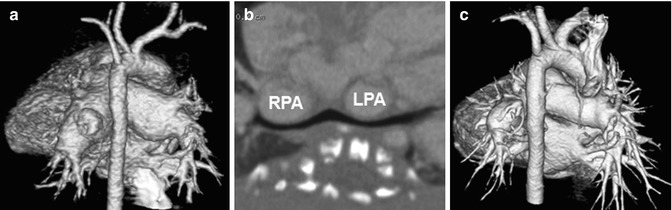
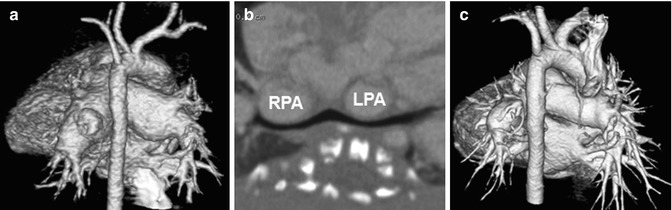
Fig. 18.9
Absent pulmonary valve syndrome. (a) Volume-rendered CT image with a posterior view shows aneurysmal dilatation of central pulmonary arteries. (b) Curved planar reformatted CT image along the bronchi demonstrates bilateral bronchial compressions by dilated central pulmonary arteries. (c) Volume-rendered CT image with a posterior view shows substantial decrease in size of dilated central pulmonary arteries after reduction pulmonary arterioplasty. RPA right pulmonary artery, LPA left pulmonary artery
In pulmonary artery sling, an aberrant left pulmonary artery arises from the proximal right pulmonary artery, courses between the trachea and the esophagus, and extends to the left pulmonary hilum. In addition to this anomalous course of the left pulmonary artery, cardiac CT can show associated airway anomalies, such as tracheal bronchus, bridging bronchus, congenital tracheal stenosis, and tracheomalacia [72–75]. Rarely, one of the two pulmonary arteries arises aberrantly from the ascending aorta and the other arises normally from the main pulmonary artery. Anomalous origin of the right pulmonary artery is far more frequent than the left one.
Pulmonary Vein
A part of (partial anomalous pulmonary venous return, PAPVR) or all (total anomalous pulmonary venous return, TAPVR) pulmonary veins may show anomalous drainage into a systemic vein, the right atrium, the coronary sinus, or the portal vein [76–79]. Exact sites of drainage and obstruction in TAPVR or PAPVR should be accurately identified before surgery, which can be better demonstrated on cardiac CT than on echocardiography (Figs. 18.10 and 18.11). TAPVR is classified as supracardiac (50 %), cardiac (25 %), infracardiac (20 %), or mixed (5 %) type according to anomalous drain sites. Infracardiac type is almost always obstructive due to an extrinsic narrowing by the diaphragm or pulmonary venous drainage through the hepatic sinusoids. Right-sided PAPVR is frequently associated with the sinus venous type of ASD [80] (Fig. 18.11). In addition, PAPVR is a component of scimitar syndrome and horseshoe lung is rarely associated with scimitar syndrome [81, 82].
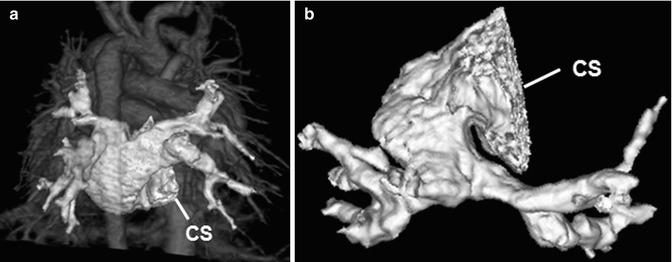
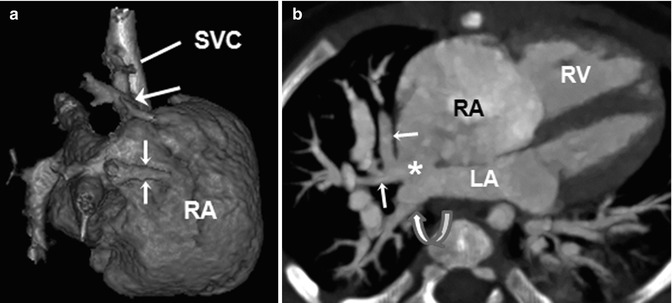
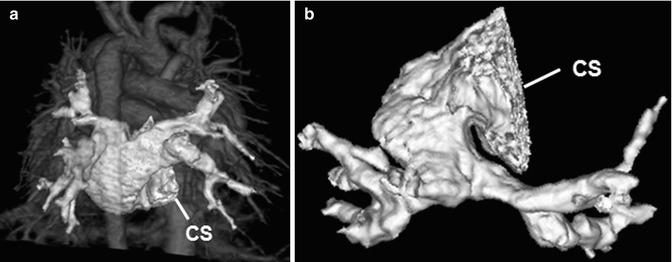
Fig. 18.10
Total anomalous pulmonary venous return (TAPVR). Volume-rendered CT image with a posterior view (a) and an inferior view (b) shows a cardiac type of TAPVR in which all pulmonary veins anomalously drain into the dilated coronary sinus (CS)
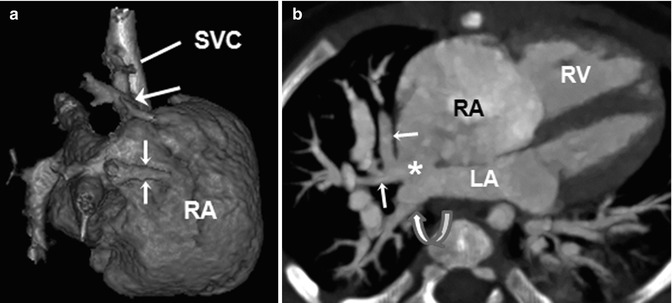
Fig. 18.11
Partial anomalous pulmonary venous return (PAPVR) and the sinus venous type of atrial septal defect (ASD). (a) Volume-rendered CT image with a right lateral view shows the right upper lobe vein (long arrow) and the right middle lobe vein (short arrows) anomalously draining into the superior vena cava (SVC) and the dilated right atrium (RA), respectively. (b) Four-chamber CT image reveals the sinus venous ASD (asterisk). The right middle lobe vein (arrows) anomalously drains into the RA, while the right lower pulmonary vein (curved arrow) normally drains into the left atrium (LA). RV right ventricle
Congenital pulmonary vein stenosis and hypoplasia/atresia may involve individual or multiple pulmonary veins [77]. The abnormalities may be focal or diffuse and unilateral or bilateral. Anatomic details of these abnormalities can be more accurately and promptly evaluated with cardiac CT than with echocardiography, and cardiac CT is, therefore, a viable alternative for invasive conventional catheter pulmonary venography [83, 84].
Systemic Vein
Persistent left SVC is frequently seen in patients with congenital heart disease [85]. A small or large bridging vein is present between bilateral SVC in approximately 30 % of cases. In about 90 % of cases, a persistent left SVC drains into the right atrium via the dilated coronary sinus [86
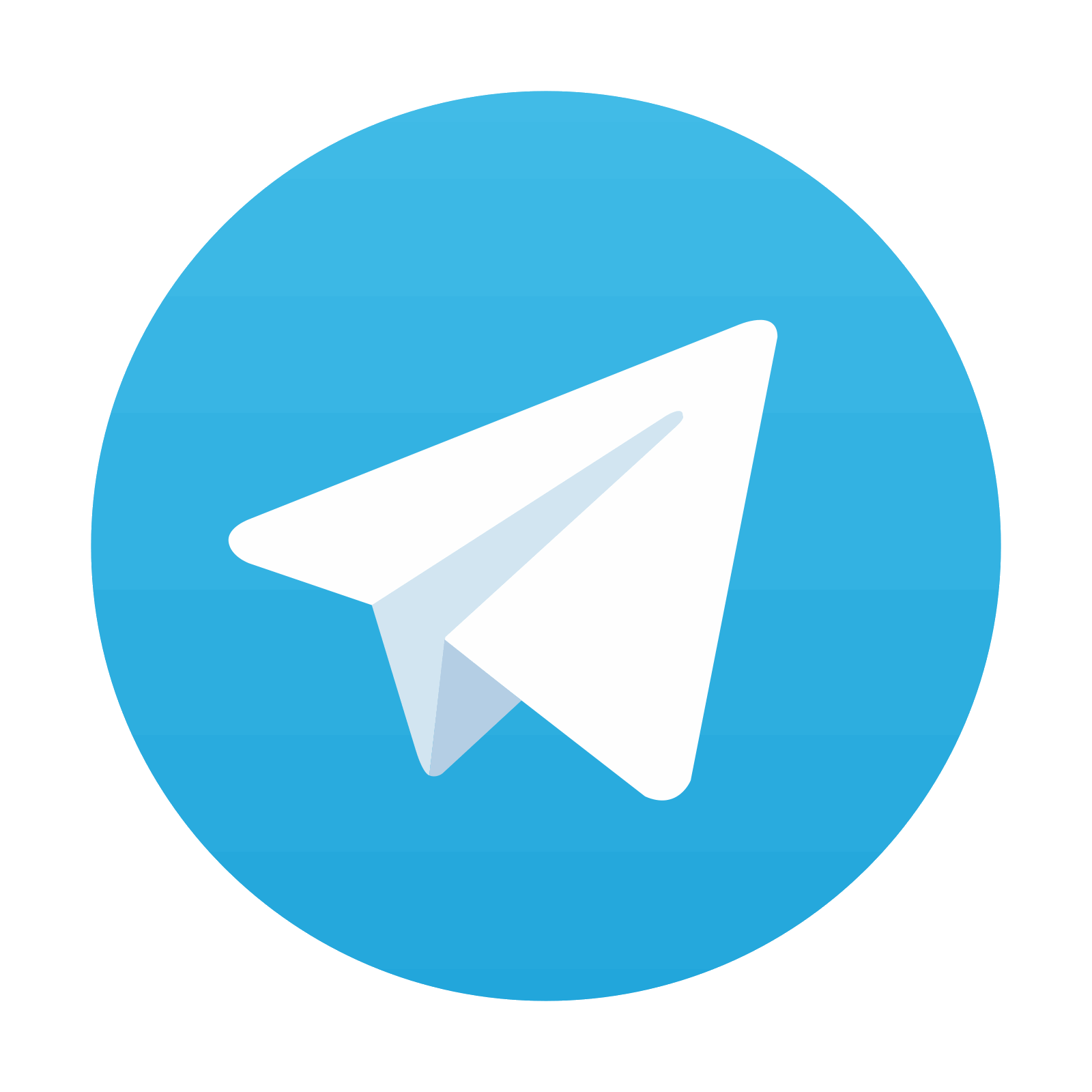
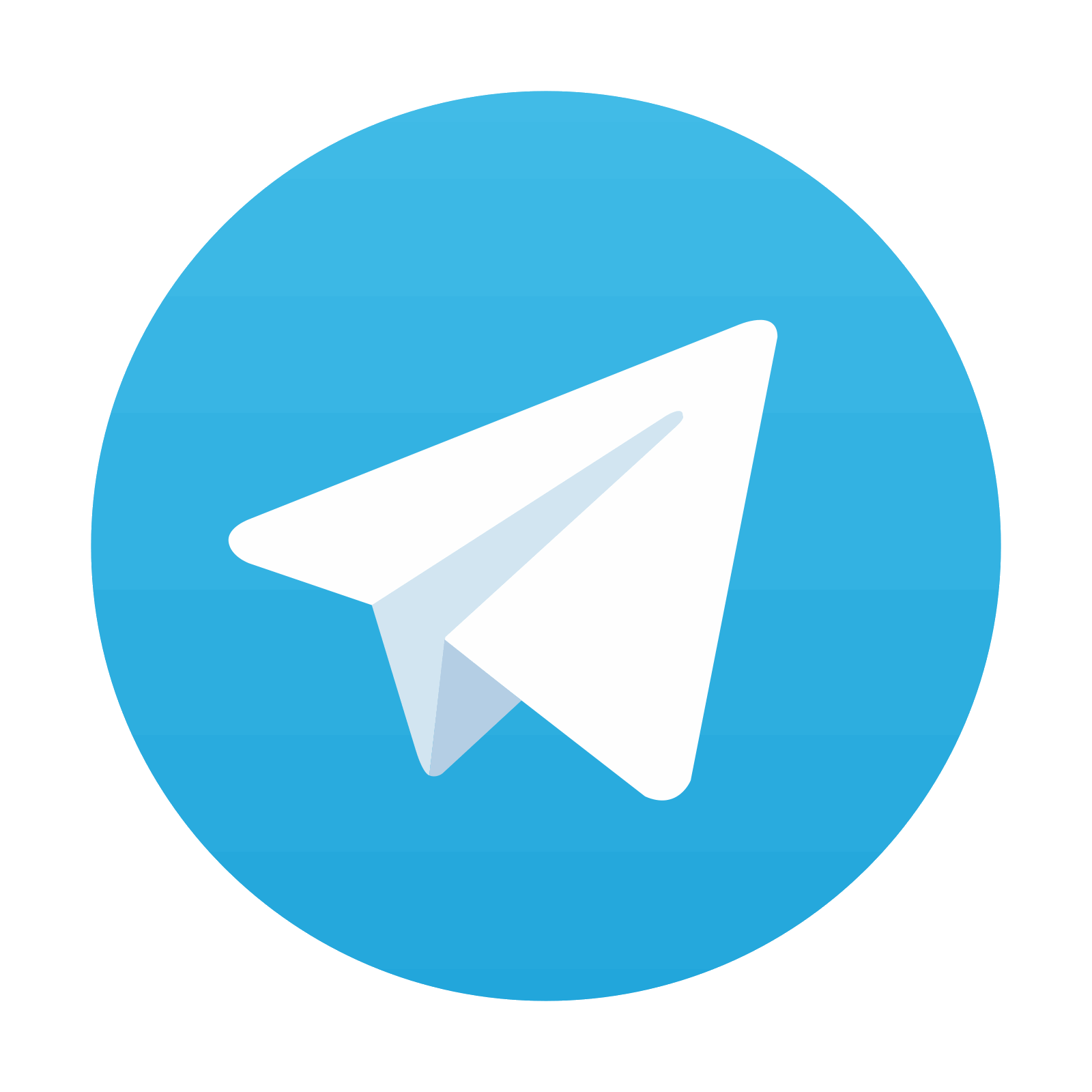
Stay updated, free articles. Join our Telegram channel
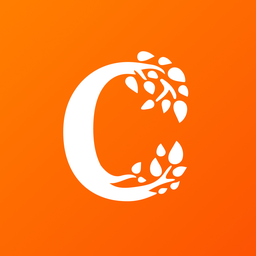
Full access? Get Clinical Tree
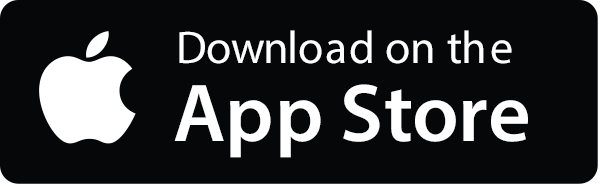
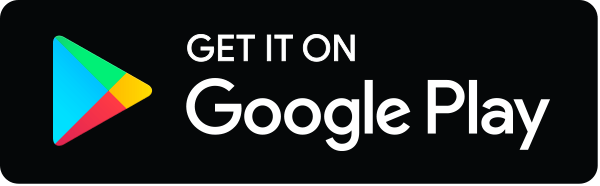