Fig. 3.1
Example of a native coronary artery (CAC) scan acquired by multidetector CT in a 62-year-old male asymptomatic subject. (a) Three-dimensional volume-rendered display demonstrating the distribution of the calcified atherosclerotic plaque in the right coronary artery (arrow). (b, c) Axial reconstructions used for quantification of CAC demonstrating extensive CAC in the left anterior descending coronary artery and the distal segment of the right coronary artery (arrow, b and c, respectively). AA ascending aorta, LA left atrium, and LV left ventricle. The Agatston score was 452 in this subject, which puts him on the 57th percentile of his matched age and gender group
3.2.1 Pathophysiological Correlate and Clinical Problem
Subclinical coronary calcification commonly occurs early in the development of atherosclerotic plaque, preceding the onset of clinical coronary heart disease by years or even decades, therefore serving as an ideal biomarker depicting the degree of atherosclerosis. Calcium is not present in normal arterial vessel walls but is present in atherosclerotic vessels. Previous studies indicate that the amount of calcified atherosclerotic plaque is highly related to the overall plaque burden and is thought to be present in the advanced stages of atherosclerosis [2]. Conversely, noncalcified plaque is considered to be a feature of early atherosclerosis [3] and may be associated with acute coronary syndromes [4]. The pathophysiologic process leading to coronary calcification is a complex one and centrally mediated by inflammation. Current evidence suggests that atherosclerosis is a systemic chronic inflammatory condition involving multiple vascular distributions, associated with a variety of clinical sequels [5]. Through a process of plaque vulnerability, rupture, and subsequent thrombosis, subclinical end-organ damage occurs, as well as clinical evident morbidity and mortality.
3.2.2 Methodological Approaches and Distribution in Normal Cohorts
With the advent of CT scanners with ECG-triggered acquisitions in the late 1990s, electron beam CT (EBCT) followed by ≥4 slice multidetector CT enabled measurement of CAC within a single breath hold [6]. The high-density contrast between calcification and adjacent tissue allowed for quantification of CAC, despite that the detection and quantification of noncalcified plaque and coronary stenosis on CT angiography (CTA) remained challenging using these first scanner generations.
Given the superb contrast induced by coronary calcification, scans are acquired without the administration of iodinated contrast agents. Traditionally, these scans are associated with a radiation dose between 1 and 2 mSv but lowered to even below 1 mSv when applying a tube voltage of 100 kVp rather than 120 kVp. Recently, additional radiation dose-reduction strategies based on iterative reconstruction have been introduced, resulting in radiation dose reductions of up to 80 % with an effective radiation dose between 0.15 and 0.18 mSv [7].
Initially developed for EBCT, the “Agatston” score, remained the traditional biomarker of CAC as a semiquantitative algorithm based on measurements of plaque area and a weighted plaque density score [8]. This results in a range of continuous values ranging from no calcification (consistent with a zero Agatston score) to extensive calcification that may add up to an Agatston score of more than 3,000. Due to the early implementation in large asymptomatic cohort studies, a normal distribution of the Agatston score with respect to age and gender became available [9]. This allowed for the determination of the percentile distribution of an individual Agatston score with respect to an age- and gender-matched reference cohort and the definition of risk categories [10]. One prominent example is the Multi-ethnic Study of Atherosclerosis (MESA), a large US study that allows to obtain the estimated probability of nonzero calcium, as well as the 25th, 50th, 75th, and 90th percentiles of the CAC distribution for a particular age, gender, and race (http://www.mesa-nhlbi.org/calcium/input.aspx) [11].
3.2.3 Scientific Evidence of Coronary Calcium Score as a Diagnostic and Prognostic Biomarker
Despite its early establishment, CAC scoring was only accepted as a screening tool for asymptomatic subjects within the cardiovascular community after strong data on its prognostic value had been accumulated. In a comprehensive meta-analysis pooling four major studies on the prognostic value of coronary calcification, Pletcher et al. found an adjusted relative risk of death or myocardial infarction of 2.1 for a CAC score of 1–100 compared to a score of 0 [12]. In addition to its generally strong prognostic value, there is similarly high level of evidence that this prognostic value is independent of other markers of risk. For instance, in the MESA study, CAC was compared to high-sensitive CRP, carotid intima-media thickness, ankle brachial index, brachial flow-mediated dilation, and family history of CHD in 1330 individuals without diabetes mellitus [13]. While each of the individual markers was predictive as indicated by an improvement of the area under the receiver-operator characteristic curve (AUC) over a clinical risk score (Framingham Risk), coronary calcification was the strongest predictor of risk associated with the highest increment of the AUC. As a consequence, the 2010 American College of Cardiology/American Heart Association (ACC/AHA) guidelines on screening for coronary artery disease indicated that measurement of CAC is reasonable (level of evidence B) for cardiovascular risk assessment in asymptomatic adults at Framingham intermediate risk (10–20 % 10-year risk) [14].
While the prognostic value in asymptomatic patients is certainly dominating the clinical role of CAC as an established imaging biomarker, there are research findings from the early years documenting a moderately valuable diagnostic role for the detection of significant coronary stenosis. For instance, in a review of 16 studies, the sensitivity and specificity of EBCT for the detection of coronary stenosis were 91 and 49 %, respectively [15]. However, despite these very nonspecific findings, the absence of CAC is highly predictive of the absence of significant stenosis (<1 %) [16]. With the advent of coronary CT angiography, the diagnostic role of coronary artery calcification scanning has diminished, given that even in subjects without coronary calcification, a portion has a plaque and a significant coronary stenosis (13 % and 3.5 %, respectively) [17].
3.2.4 Future Outlook and Developments
Today, assessment of coronary calcification by CT is a highly established biomarker of cardiovascular risk in asymptomatic subjects. However, one of its limitations is that the effectiveness of implementing CAC scanning in therapeutic algorithms has not been established yet in randomized trials. So far, very early evidence stems from the St. Francis Heart Study, in which healthy men and women with CAC scores ≥80th percentile for age and gender were treated with atorvastatin, vitamins C and E, or matching placebos [18]. However, there was only a nonsignificant trend toward a lower rate of cardiovascular events. Due to these limited and nonsignificant findings, pharmacologic therapy to prevent CHD based solely upon the presence of CAC is currently not recommended.
3.3 Imaging Biomarker of Myocardial Fibrosis: Late Gadolinium Enhancement MRI
Up to now, cardiac biomarkers in clinical routine have been dominated by protein molecules that are widely used in the (early) detection of heart failure. For example, serum levels of troponin T (TnT), C-reactive protein (CRP), and brain natriuretic peptide (BNP) have been shown to be sensitive markers of left ventricular (LV) dysfunction and powerful markers of morbidity and mortality in the heart failure setting. One of the current methods of monitoring LV function in patients suffering from heart failure is determination of left ventricular ejection fraction (LVEF) with echocardiography. However, assessment of LVEF is dependent on hemodynamic conditions and fails to detect early subtle alterations in LV systolic function that occurs in the later stages of chronic disease. In addition to echocardiography, cardiac magnetic resonance imaging (MRI) might be used for the noninvasive assessment of LV volumes and LVEF in a heart failure setting [19]. Improvements in both spatial and temporal resolution have made cardiac MRI the gold standard for the noninvasive assessment of LV systolic function, also in patients after myocardial infarction [20].
3.3.1 Clinical Problem
One of the main advantages of cardiac MRI over other imaging modalities is its ability to qualitatively and quantitatively assess changes in myocardial tissue. For example, by using contrast-enhanced pulse sequences, cardiac MRI can be used to assess myocardial viability. Moreover, in myocarditis, this has been used to assess edema, capillary leakage, hyperemia, and, in severe cases, cellular necrosis and fibrosis. This technique has displayed high diagnostic accuracy for acute inflammatory or ischemic injury, which is significant as edema is an important hallmark of inflammatory injury. Nowadays, late gadolinium enhancement (LGE) imaging has become the primary diagnostic tool for assessment of myocardial viability in patients suffering from CAD as well as myocardial inflammation in patients with suspected myocarditis of cardiomyopathies [21]. Furthermore, cardiac stress MRI using adenosine might add a functional component to late gadolinium enhancement imaging, which detects irreversibly damaged myocardium (e.g., scar tissue) with very high accuracy. With LGE, hypo- or akinetic but still viable myocardium can be identified as dysfunctional myocardium without scar or significant remaining viable tissue (<50 % transmurality of scarring). In clinical practice, this additional information will help to decide whether or not the patient will recover after revascularization. However, different noninvasive tests provide different surrogate definitions of hibernating myocardium (e.g., metabolism, scar, or contractile reserve), and consequently the result of a given investigation may depend on the chosen imaging test.
3.3.2 Pathophysiological Correlate
The mechanism of LGE in acute and chronic infarction is the increase of the extracellular space caused by necrosis (loss of cell membrane integrity), resulting in a significant increase of the extracellular space of scar tissue as compared to normal myocardium. Magnetic resonance contrast agent that diffuse to the interstitial space will be resorbed into the capillary bed and undergo renal excretion. However, when the tissue is damaged, for example, due to infarction, diffuse fibrosis, or even inflammation, the resorption rate of contrast agent will be diminished. At 10–20 min after contrast injection, washout of the contrast agent will be complete in normal myocardium, in contrast to infarcted or edematous tissue. This phenomenon is the basis of “late gadolinium enhancement” imaging. Various studies have shown the relation between myocardial viability and the size of the area displaying late gadolinium enhancement. In MR images, the presence of contrast agent can be detected as a bright area on images acquired with T1-weighted MR images. Currently the principle “bright is dead,” indicating that bright areas on a late gadolinium MR image after contrast injection correspond with nonviable myocardium, is subject to a lively debate. Nevertheless, a strong correlation between the transmural extent of hyperenhancement and regional function recovery has been demonstrated in several studies, revealing LGE imaging as a powerful predictor of myocardial damage after myocardial infarction.
The mechanism in nonischemic myocardial diseases is also predominantly, if not exclusively, due to increase in the distribution volume of gadolinium, caused by an increase in the extracellular space of the myocardium. The increase in the extracellular space may be due to necrosis of myocardial cells (myocarditis), replacement of myocardial cells by fibrosis (hypertrophic cardiomyopathy), or replacement of myocardium by various infiltrates (e.g., amyloidosis, sarcoidosis), or a combination of these.
While the distribution of LGE is invariably subendocardial for ischemic disease (either acute or chronic myocardial infarction), the pattern of enhancement has a variable transmural distribution in nonischemic myocardial disease. LGE conforms to the distribution of one or more coronary arteries in ischemic heart disease, while in nonischemic myocardial disease, it does not. With nonischemic myocardial disease, the mural pattern usually can be seen midwall or subepicardial and may be spotty in multiple regions of the ventricle. The pattern of distribution in some may be almost diagnostic of a specific myocardial disease.
3.3.3 Scientific Evidence and Prognostic Impact
Within the bright LGE area of an acute myocardial infarction, microvascular obstruction (MO) or the “no-reflow” phenomenon is known as an established complication of coronary reperfusion therapy, adding another complementary “imaging biomarker” to the clinical value of LGE (Fig. 3.2) [22–24]. The phenomenon of microvascular obstruction is increasingly recognized as a poor prognostic indicator and can serve as an imaging marker of subsequent adverse LV remodeling [25]. Although MO can be assessed using various imaging modalities, evaluation by cardiac MRI is particularly useful in enhancing its detection, diagnosis, and quantification, as well as following its subsequent effects on infarct evolution and healing. MO assessment has become a routine component of the MR evaluation of acute myocardial infarction and will play an increasingly important role in therapeutic decision pathways. MO is characterized by a number of ultrastructural and functional changes at the microvascular level. Understanding these histopathophysiologic changes can enhance the approach by MRI to detecting MO, to understand the results and their subsequent clinical implications. It can also help to potentially improve how MO is assessed by CMR, which has implications with regard to the understanding of infarct evolution as well as the evaluation of the efficacy and mechanism of reperfusion treatment.
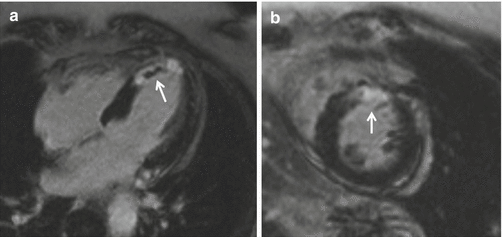
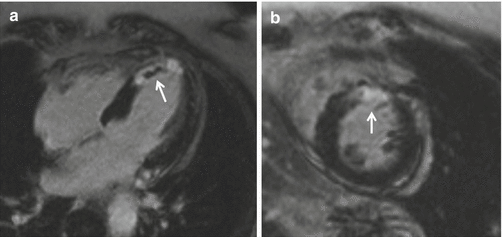
Fig. 3.2
A 77-year-old female patient suffering from an acute myocardial infarction. Invasive catheter coronary angiography showed complete occlusion of the left anterior descending artery (LAD), with following revascularization of the LAD territory after successful PTCA and stent placement. In the four-chamber view of the late gadolinium enhancement (LGE) image (a), a myocardial infarction can be seen by pathological uptake of the contrast agent (arrow) and with an area of microvascular obstruction in the center of the infarcted myocardium (black central area within the LGE area). Short-axis late gadolinium enhancement image through the mid-ventricle at the level of the papillary muscles (b) demonstrates the corresponding myocardial delayed enhancement in the anterior-septal wall segments (arrow)
LGE and MO have been found to be predictive of clinical outcome, independently of or when adjusted for other indices such as infarct size and LV ejection fraction (EF) [26]. Many of these outcome studies showed a severe relationship between the size of LGE and/or the presence of MO and adverse LV remodeling, with reduced global systolic function and pathologically enlarged LV volumes at follow-up exams, suggesting a possible mechanism for the poor prognosis. Moreover, MO is increasingly incorporated into clinical trial methodology as a surrogate clinical outcome in studies investigating reperfusion strategies, particularly when the treatment has the potential to directly impact microvascular function.
3.4 Imaging Biomarker of Tumor Angiogenesis: Assessment of Perfusion
Angiogenesis is a complex process and a constant companion of normal tissue development and tissue formation but shows certain alterations in pathologic processes like tumor growth and/or inflammatory processes. Perfusion-based imaging enables objectification and quantification of angiogenesis and thus opens insights into a functional process generating surrogate biomarkers that are complementary to, e.g., morphologic tumor imaging. The knowledge that angiogenesis is a prerequisite for tumor growth and tumor spread makes this biomarker a plausible target not only for tumor and tissue characterization purposes but also for prognosis evaluation and treatment monitoring. In case of inflammatory processes, perfusion-based imaging allows indirect assessment of inflammatory activity which is usually going along with an increased tissue perfusion and offers potential tool for monitoring anti-inflammatory therapy regimens.
The most frequently used parameters, or quantitative imaging biomarkers of tissue perfusion, are the blood flow (the rate of blood passing through the vasculature in a tissue region), the blood volume (the volume of blood that is actually following within the vasculature), and the mean transit time (the average time of blood traversing from the arterial input to the venous outlet and k-trans (a surrogate measure of vascular leakiness reflecting the flux of solutes from blood plasma to the interstitial space)). Each of these parameters represents a facet of the complex process of angiogenesis and can be used for tumor characterization, for understanding of the mechanisms of action of molecular (antiangiogenic) therapeutic agents, and thus for a potentially more sensitive response monitoring (early detection of response and/or early recognition of tumor breakthrough) (Fig. 3.3).
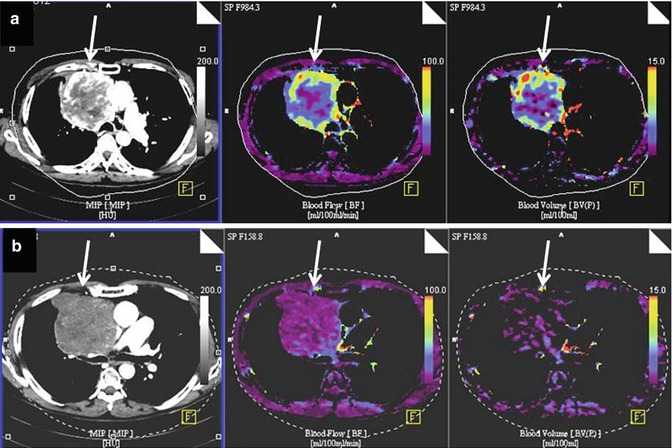
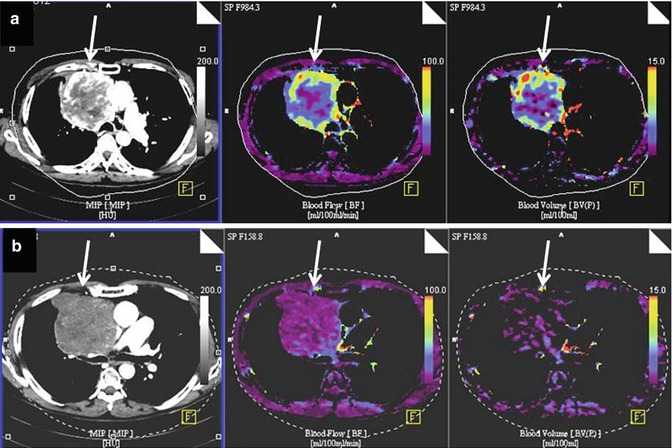
Fig. 3.3
Solitary fibrous pleural tumor (Panel a and b, arrows), receiving experimental therapy with bevacizumab (VEGFR inhibitor) and temozolomide as sixth-line treatment attempt. Perfusion CT performed at baseline (upper panel, a) shows high levels of tumor perfusion (from left to the right morphologic image/blood flow [BF] color-coded map/blood volume [BV] color-coded map) at the tumor edges with large core necrosis. Six weeks later, during ongoing therapy, a typical on/off angiogenic switch could be observed (lower panel, b)
3.4.1 The Clinical Problem and Pathophysiological Correlate
Recent developments in tissue characterization in terms of histological differentiation, grading, but in particular immunohistochemical profiling (i.e., receptor expression) as well as genome typing, needing invasive procedures such as biopsies, have somewhat interfered with the constant ambition of establishing and optimizing imaging biomarkers for noninvasive tumor characterization. Nonetheless, relevant clinical applications focusing on perfusion-based tumor identification and characterization are being developed and constantly enhanced. One of the most important clinical applications for perfusion imaging is the characterization of hepatic nodules occurring in patients with known liver cirrhosis, fatty liver, hepatitis C, or hemochromatosis. In these cases, current diagnostic guidelines recommend a noninvasive HCC diagnosis based on the presence of typical enhancement patterns like washin and washout phenomena, in contrast-enhanced CT or MR imaging. Unfortunately, these typical qualitative enhancement patterns are not always present in HCC nodules, and potentially being missed, in particular in smaller lesions. In this clinical setting with unclear imaging results, an invasive approach, i.e., biopsy, is usually recommended. The rationale for implementing a quantitative, perfusion-based imaging biomarker of HCC and of HCC precursors is the knowledge that along the so-called multistep process of carcinogenesis, liver nodules create an arteriolar network, gradually replacing the normal sinusoidal architecture and thus becoming predominantly or exclusively supplied by arterial blood [27]. Hence, with ongoing tumor differentiation, the number of so-called non-triadal arteries is increasing which has been intensively analyzed by comparison with histology [28]. At this point, the use of perfusion imaging (e.g., perfusion CT) with the option for a separate calculation of the dual liver blood supply (arterial and portal venous blood supply) offers an ideal tool for a noninvasive classification of liver lesions [29]. Additionally, differences in the perfusion between parenchymatous organs like the liver and metastases are known to improve tumor detection both for hypervascularized and hypovascularized lesions, based on the fact that all these lesions are primarily supplied by arterial blood [30]. In several tumors, a direct correlation between them and other invasive histological biomarkers of angiogenesis like microvessel density and VEGF values has been reported [31, 32]. Despite the need for a histologic proof in the primary diagnosis, detection of tumor relapse could benefit from noninvasive imaging-based characterization, avoiding unnecessary risks related to invasive diagnosis. In particular in anatomical region like the CNS, avoidance of open biopsy has emerged to an issue of great debate. A number of studies have used perfusion CT for tumor grading showing that BF, BV, and k-trans values were higher in high-grade glioma vs. low-grade glioma [33]. Moreover, differentiation of high-grade gliomas from other brain lesions including lymphomas has been reported based on differences in BF and BV [34].
3.4.2 Perfusion Imaging as a Biomarker for Prognosis and Response
Lately, perfusion parameters have been increasingly used to predict overall survival after therapy or to predict response to different treatment regimens [35]. Some authors reported about the potential association between the degree of tumor vascularization and tumor aggressiveness [36]. An increased local failure rate was reported in squamous cell carcinoma of the head and neck presenting with low perfusion values before treatment [37]. Concordantly, a better response to both chemotherapy and radiation therapy was described in tumors exhibiting higher BF values in the baseline [38]. Early efficacy prediction of sorafenib in the treatment of hepatocellular carcinoma (HCC) could be demonstrated in rats [39]. Pretreatment perfusion values were found to be a good predictor in many other tumors undergoing antiangiogenic therapy [40].
A response biomarker may be defined as a characteristic that can be objectively measured as an indicator of pharmaceutical response. The way and magnitude by which tumor vascularization is affected by a certain drug depends both on the characteristics of the tumor’s own vessel network, that of its microenvironment as well as on the type of antitumoral agent applied. Knowledge about all these variables helps for adequate use of perfusion measurements considering any potential clinical setting. In the long term, most effective therapy regimens lead to a reduction in perfusion CT parameters. Even during standard chemotherapy, cell death is expected to be followed by a loss in angiogenic support leading to measurable hypovascularization. In particular, of interest are the angiogenesis inhibitors which in many cases are administered as monotherapy. Their antiangiogenic effects depend on the mechanism of action of the drug applied as well as on the specific tumor entity, whereas morphologic changes are generally not expected at least in the early phases of treatment. Despite concurrent imaging modalities including positron emission therapy using different radiopharmaceuticals, targeting the course of tumor vascularization seems to be the more accurate way of response assessment during antiangiogenic treatment. Moreover, perfusion parameters are also suited for confident response monitoring for both local antivascular therapies like chemoembolization and systemic treatment. Even during radiation therapy, changes in perfusion characteristics can be predictive for progressive-free survival and outcome [41]. Perfusion CT also enhances our understanding of the pharmacodynamics of novel drugs and how they should be combined with each other in order to achieve best therapeutic effects. In this respect, one good example is the class of vascular disrupting agents that target also the mature vasculature potentially impacting the arrival time and dose of other complementary-given chemotherapeutics. Using perfusion as a complementary biomarker helps for developing new more proper response criteria, avoiding confusion caused by newly described response categories like pseudoresponse, pseudostabilization, or even pseudoprogression, which severely affect patients’ management [42]. Many of the so-called targeted drugs directly or indirectly affecting the signaling pathways of angiogenesis, like EGFR, VEGFR, and PDGF inhibitors, interferon, proteasome inhibitors, and thalidomide and successor drugs, have already been extensively analyzed by means of perfusion imaging techniques [43, 44].
In conclusion, perfusion-based imaging is a potent imaging tool which requires profound understanding of tumor behavior and mechanisms of action of the drugs to be monitored. Once these aspects have been elucidated, its broad clinical use may be advocated.
3.5 Imaging Biomarker of Osteoarthritis: T2 Relaxation Time of the Articular Cartilage
3.5.1 Clinical Background
Osteoarthritis is a common degenerative joint disease, affecting more than 20 million people in the USA alone. The incidence of osteoarthritis is rising, and in 2030, at least 70 million people (USA) will be affected [45]. Osteoarthritis-related costs are a relevant socioeconomic factor. The established therapy of manifest osteoarthritis is cartilage repair, cartilage transplantation, or arthroplasty, while lifestyle modification is currently the only method of potential disease prevention [46]. Disease-modifying therapies which may prevent or prolong the course of osteoarthritis have been developed and evaluated but are still not broadly approved for clinical routine.
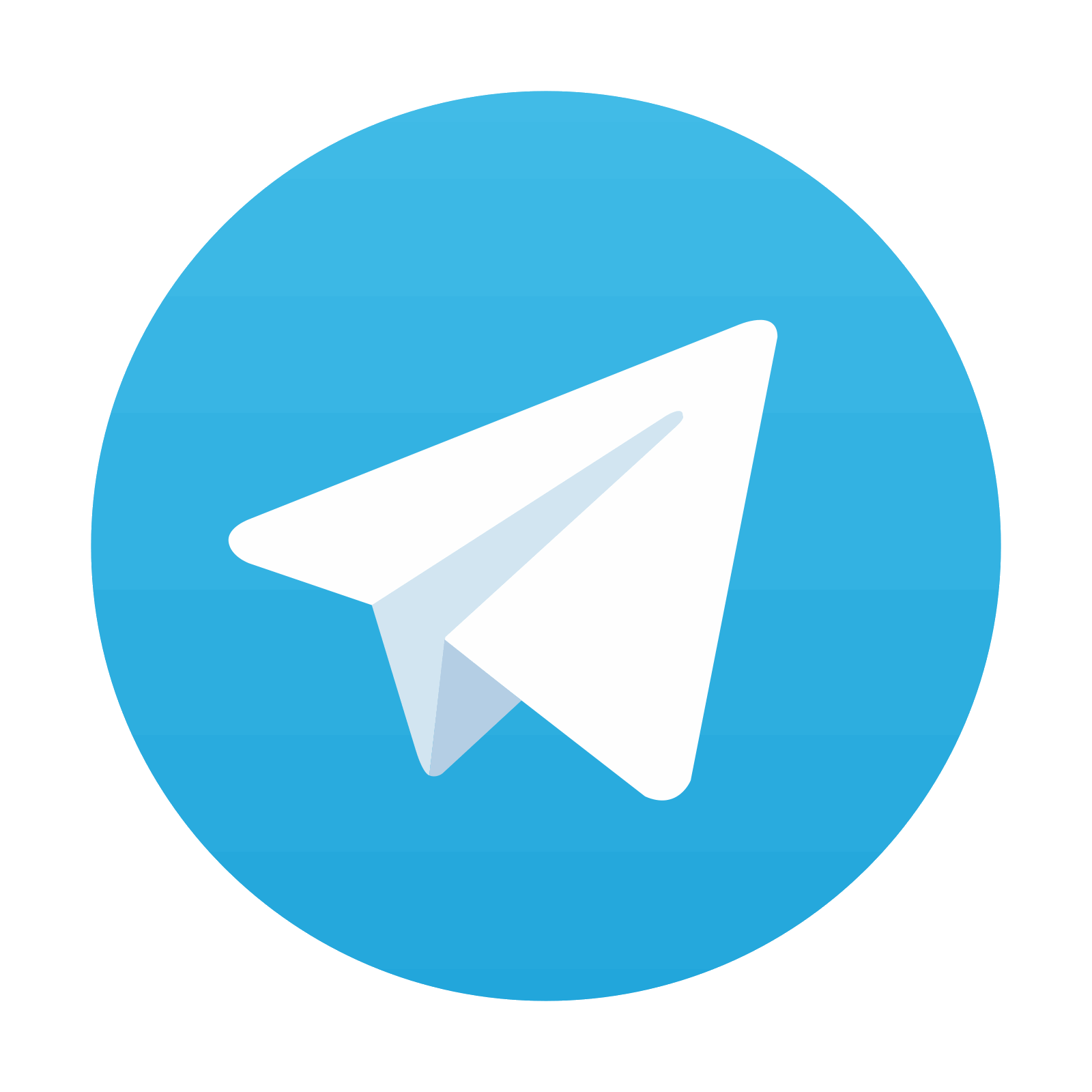
Stay updated, free articles. Join our Telegram channel
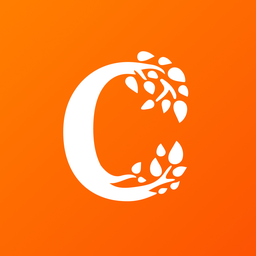
Full access? Get Clinical Tree
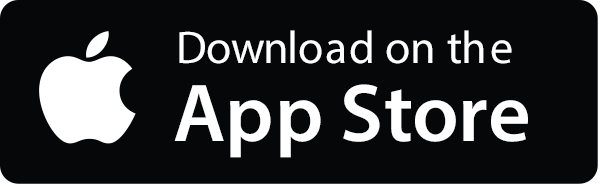
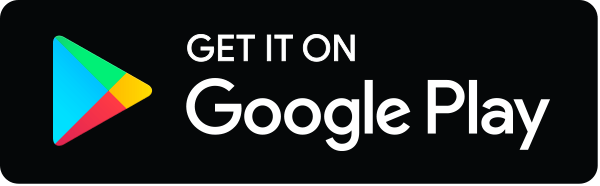