Fig. 11.1
Illustration of the physical mechanisms underlying the biological effect of soft or hard shell Ultrasound Contrast Agents (Adapted from Kiessling et al. 2014)
11.2.3 Drug Delivery with UCA and Ultrasound
11.2.3.1 Plain UCA with Free Drug
There are several reports describing the use of a mixture of UCA and drug for therapeutic purposes. This is a very convenient approach due to the immediate availability of both microbubbles and drug agents. It is also seen as a straightforward continuation of the current diagnostic use of clinically approved agents. Thus, many of these reports have used marketed UCA (Definity®, SonoVue®, Optison®…), and most advanced clinical studies have been conducted for the purpose of clot lysis, so-called sonothrombolysis (de Saint Victor et al. 2014), and more recently for pancreatic cancer (Kotopoulis et al. 2014). Conditions described were in presence or absence of fibrinolytic agent r-tPa, and under the regime of various ultrasound settings (various frequencies, acoustic pressure, pulse repetition frequency…). Some clinical trials have shown the potency of this approach to treat patients suffering from stroke by enabling faster and more complete recanalization (Molina et al. 2006). However, current trials did not provide significant improvement in patient conditions at 3 months post-treatment, partially due to the limited number of patients enrolled. Moreover, one of the main lessons to retrieve from these studies is that still little is known of the mechanism underlying UCA-enhanced sonothrombolysis (STL), and in some cases, lack of control of the biological effects of this new therapeutic approach has triggered some safety concerns. It is thought that design of UCA specific for STL indication has the potential to further improve not only their effect as treatment enhancers, but also their safety profile.
The approach of administering a mixture of UCA and drug has also been exploited for other therapeutic applications, such as delivery of cytotoxic drug (Ibsen et al. 2013a; Escoffre et al. 2013), BBB opening (Aryal et al. 2014) or gene delivery (Rychak and Klibanov 2014; Newman and Bettinger 2007). The recent report of a successful chemotherapy improvement by using both ultrasound and UCA to treat pancreatic cancer has highlighted the clinical feasibility of such an approach, even though this has only been done on a limited number of patients (Kotopoulis et al. 2013).
In parallel to the co-administration approach of free drug and UCA, several groups have also investigated the potential of co-localization of drug and cavitational activity on therapeutic efficiency by preparing drug-loaded UCA. Indeed, it is speculated that incorporation of therapeutic agent close to the UCA shell would be more favorable since cavitation will likely drive delivery.
11.2.3.2 Drug-Loaded UCA
Drug loaded UCA have been formulated using different approaches, as illustrated in Fig. 11.2. Several strategies have been employed to incorporate therapeutic agent in UCA, such as chemical conjugation, electrostatic adsorption at the outer surface and embedding in the shell. The choice for the best method of loading depends mainly on the nature of the drug.
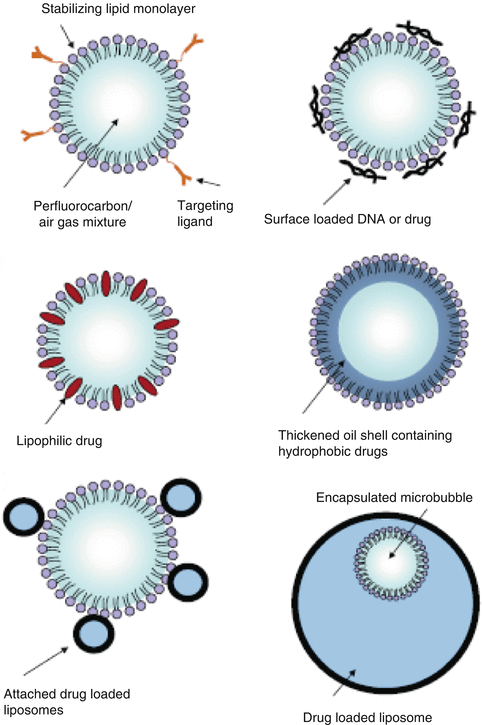
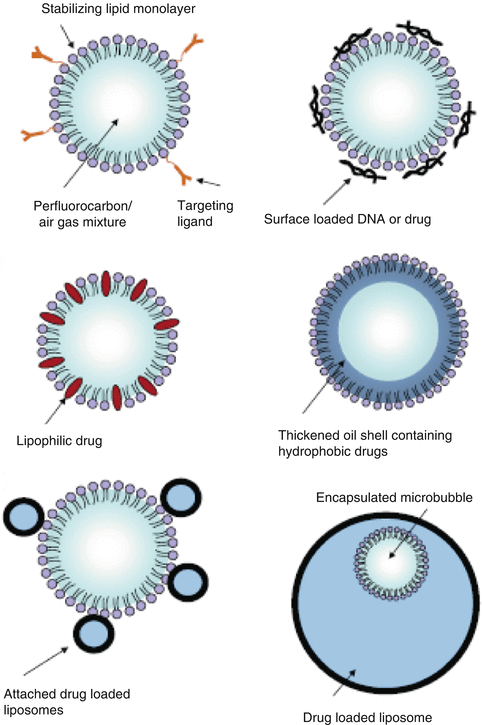
Fig. 11.2
Various options for the design of drug-loaded ultrasound contrast agent. (a) The basic microbubble design with a lipid monolayer stabilizing the gas core. Targeting ligands can be conjugated to the surface to help facilitate accumulation of the microbubble in desired tissues. These ligands can be antibodies or short peptide sequences. (b) The microbubble itself can be a vehicle by attaching drugs and even DNA to the surface through the use of electrostatic attractions. (c) Lipophilic drugs can be incorporated into the lipid monolayer shell of the microbubble. (d) The stabilizing shell can be thickened with an oil layer, allowing hydrophobic drugs to be carried within it. (e) Drug-filled liposomes can be attached to the surface of the microbubble. When the microbubble is exposed to ultrasound, the liposomes are disrupted by the mechanical actuation of drug release. (f) The microbubble can be encapsulated within a liposome along with the drug. When exposed to ultrasound, the microbubble ruptures the outer liposome, releasing the payload (Reproduced from Ibsen et al. 2013a)
One additional advantage of loading UCA with therapeutics is that it can act as a protective drug carrier. Thus, unstable drugs can be protected from degradation in biological fluids, thus prolonging their half-life. This was nicely illustrated by DNA molecules, for which enhanced resistance to nuclease degradation was measured (Lentacker et al. 2006). On top of that, the limited release outside the insonified area due to the relatively low peripheral microbubble destruction could help in preventing major adverse events related to the drug itself.
11.2.3.2.1 Drug in/on the Shell
Some studies have demonstrated that covalent attachment of the drug is more favorable to elicit a therapeutic effect. For example, conjugation of rose bengal onto UCA was proven to be more cytotoxic compared to un-conjugated sonosensitizer (Nomikou et al. 2012); so-called sonodynamic therapy (SDT). This approach could be of interest for the treatment of cancer lesions, and thanks to a relative good US tissue penetration, it appears better than typical light excitation used in photodynamic therapy (PDT).
However, careful evaluations are warranted to measure the impact of the loading on the actual drug activity. For example, r-tPA proved to be a quite complex molecule to formulate with the need to use specific conditions to maintain biological activity (risk of aggregation of the native protein, personal results). In addition, if chemical conjugation is employed, careful control of the conjugation process is needed to ensure that biological activity of the drug is not altered. Moreover, loading procedure could also impact the actual bioavailability of the drug. Thus, even though drug loading efficiency is obviously important, formula optimization should equally be considered to ensure a proper delivery of the active drug to the site of treatment.
Being inspired by cationic lipids or polymers used for non-viral gene delivery (so-called lipoplexes and polyplexes, respectively), some investigators have prepared UCA bearing cationic charges at the shell surface. For phospholipidic shells, this can be achieved by inserting cationic lipids (e.g., DSTAP) to trigger a positive zeta potential of the microbubbles. This significantly increases the loading of nucleic acids, and several reports have demonstrated usefulness of these UCA constructs to promote gene delivery in-vitro and in-vivo (Wang et al. 2012; Rychak and Klibanov 2014).
Due to the effective loading capacity being restricted to the UCA shell, drug-loading vehicles have mainly been developed with highly potent drugs, such as nucleic acid, known to be active in the μg range. It was estimated, on average, that loading capacity of UCA for nucleic acid complexation is typically in the range of 0.01 pg/μm2. For a 2 μm UCA diameter, this corresponds to ca. 0.12 pg/microbubble. Thus, almost 10 million UCA are required to carry 1 μg of nucleic acid, illustrating the limited loading capacity of regular cationic UCA. As an example, the situation is similar for drugs when compared to usual circulating drug concentration during chemotherapy. This is why some groups have used the approach of pre-loading drug into nanoparticles prior to coupling them onto the UCA shell surface (Mullin et al. 2013).
11.2.3.2.2 Nanoparticles on UCA
These nanoparticles can be of different natures, such as liposomes (Kheirolomoom et al. 2007) or poly(lactic-co-glycolic acid) (PLGA) (Chappell et al. 2008). In particular, liposomal preparations of Doxorubicin are quite popular thanks to the availability of FDA approved products (e.g., Doxil®). Properties of the drug to be formulated are of course very important, as it will have a direct impact on the loading efficiency. For example, highly hydrophilic drugs would be difficult to embed into the shell. Most importantly, impact of the drug loading on the UCA ultrasound responsiveness must be evaluated since modifications in UCA stability, risk of aggregation and shell properties (increase in stiffness) can be observed under certain conditions.
There are numerous possible approaches to associate the nanoparticles to the UCA. This can be achieved by biotin-avidin interaction, but this approach is only valid for research or pre-clinical evaluation due to potential immune reaction with this protein (Chinol et al. 1998). An alternative mean to enable strong association relies on the use of chemical conjugation, such as amide, disulfide or thioether bond formation. Electrostatic attachment is another possibility leading to an easy preparation, but it does carry the risk of lack of reproducibility and lack of association control, impeding further development of this method towards clinical use.
11.2.3.2.3 UCA in Drug-Loaded Liposomes
Interestingly, a recent procedure has been described to increase UCA drug loading. This was accomplished by encapsulating the UCA within the internal aqueous space of a drug-loaded liposome (Ibsen et al. 2011). This rather unusual construct must be evaluated to assess not only the drug delivery potential compared to regular nanoparticle-loaded UCA, but also the US-responsiveness of the encapsulated UCA.
11.2.3.2.4 Hard Shell
In addition to the use of drug pre-formulated in nanoparticles, thick shelled UCA could also be a means to improve drug payload (Lensen et al. 2011). These agents are usually made of polymers and have shells of 20–100 nm thickness. Shell stiffness of these agents requires specific insonation parameters to mediate delivery. Indeed as these agents will not oscillate at low acoustic pressure, but require higher pressure to be activated leading to rupture and gas escape. For this reason, the potential issue that can be faced with this formulation is the drug release from the polymer shell; the shells remain in majority intact as only cracks are observed. Modification of shell properties, by altering shell thickness and composition, could improve both behaviors under US exposure and drug delivery efficiency. In addition, the nature of the polymer must be taken into consideration, particularly when dealing with safety linked to the biocomptability. In the same trend, it has been speculated that use of UCA composed of components with different shell properties can release a drug in a stepwise manner.
11.2.3.2.5 Nanoemulsion
Another possible approach is the use of drug-loaded nanoemulsion (Rapoport et al. 2009). These nanoparticles are made of liquid perfluorocarbon (e.g., perfluoropentane). US exposure triggered heating causes a droplet-to-bubble phase shift, resulting in the in-situ formation of drug loaded UCA that can be further activated by distinct US conditions. The impact of phase shift has to be addressed in detail since it can alter the chemical integrity of the loaded drug. These particles display a particularly long circulation time, with up to 50 % of the injected dose still remaining in the circulation 2 h after administration (Rapoport et al. 2011). Moreover, thanks to their relatively small size (200–500 nm), they also have the opportunity to reach compartments not normally accessible to UCA. This is by escaping the blood space, so-called extravasation. Due to the narrow temperature range required for this transition phase, the treatment could be precisely tuned by modulating this temperature increase when focusing the ultrasound beam in the specified location. This will be extensively described in another chapter of this book (see Chap. 14).
11.2.3.2.6 Monosize
Recently, a new way to formulate drug in UCA relying on the use of microfluidics technology was described. Indeed, successful preparation of multilayer gas lipospheres was reported using flow-focusing geometry (Hettiarachchi et al. 2009). These rather complex constructs are composed of a phospholipid shell, an oil layer comprising cytotoxic drug doxorubicin, and an inner gas core. Moreover, this technology can address one additional critical parameter for an efficient drug delivery; control of UCA size so that it nicely matches the selected US frequency. Thus, devices allowing UCA preparation displaying a narrow size distribution were specifically designed. These developments will likely prove to be important for further improvement of drug delivery by ensuring that all the UCA exposed to US will be activated at a defined frequency, maximizing the delivery of the loaded drug. Use of monodisperse UCA may also improve the efficacy/safety balance by allowing use of lower acoustic energy to elicit drug release, knowing that all UCA will eventually respond to US exposure at the selected frequency.
Use of UCA for BBB opening has been described in several reports (Liu et al. 2014; Choi et al. 2010), most of the tested agents being approved UCA. However, UCA formulation with a defined diameter may improve drug delivery to the brain since it was shown that 4–5 μm size was more effective than 1–2 μm UCA, under tested conditions (Choi et al. 2010). This reinforces again the need to develop manufacturing processes allowing preparation of monodisperse UCA. However, currently the main limitation is the yield of such manufacturing which does not allow a high amount of UCA preparation, meaning that acoustic characterization and in-vivo tests are still challenging.
11.2.4 Optimization of Drug Delivery
Close contact between UCA and the target tissue should intuitively favor drug delivery, vessel permeability or clot lysis. Thus, a clever way to further improve drug delivery efficiency could be the use of targeting strategies such as either passive or active accumulation. Passive targeting can result from interaction of some shell components with the specific particle’s clearance system. This is clearly illustrated by phosphatidylserine-containing UCA (e.g., Sonazoid®), reported as being efficiently taken up by Kupffer cells.
The specificity of this UCA might be increased by adopting a specific targeting strategy for it. These UCA differ from those initially developed for blood pool imaging by the presence of a targeting moiety able to link the bubble to a selected cell biomarker. The general strategy is to link molecular entities to the phospholipid-stabilizing monolayer, allowing the bubbles to remain attached to selected sites in the vascular compartment. Once attached, these bubbles act as echo-enhancers, similarly to blood pool agents, since the signal is generated by the bubble itself and not the ligand. This raised important points.
As the bubbles remain strictly within the vascular compartment, targets must be selected that are accessible to them i.e. on the luminal side of endothelial cells (Bettinger et al. 2012; Pochon et al. 2010). Two application areas are well characterized: neoangiogenesis and inflammation, since both involve endothelial cells. Tumoral neoangiogenesis (Deshpande et al. 2010; Willmann et al. 2010), i.e., the formation of new blood vessels, is a fundamental process occurring during tumor progression and is triggered by hypoxia. In the course of the inflammatory process, various cell surface markers are expressed or up-regulated on the endothelial luminal side, and are therefore accessible to targeted microbubbles. Site targeted microbubbles can also be used for the visualization of thrombi associated with stroke.
The attachment of bubbles to the surface of endothelial cells must be strong enough to withstand vascular areas where shear stress is high due to high blood velocity and viscosity. The attachment could be enhanced by adding radiation force. This improves the interactions between microbubbles themselves and their interactions with endothelial cells. Otherwise, incorporation of magnetic particles within the shell of the core could enhance attachment. By adopting such a strategy, we not only have access to highly specific areas to drive the desired therapeutic effect, but we can also significantly limit adverse events by limiting interaction with non-affected regions.
Efficient ligand-target interaction can be achieved by either using flexible spacers to present the targeting ligand in the most effective way, or by fine-tuning the ligand density at the UCA surface. To improve circulation time and binding specificity, a clever approach of a ‘buried ligand’ has also been recently proposed (Borden et al. 2006, 2008). Active accumulation could also be achieved by physical means, such as acoustic radiation force (Kheirolomoom et al. 2007; Frinking et al. 2012) or magnetic force to concentrate UCA at the site of treatment (Stride et al. 2009).
11.2.5 Characterization of UCA
An important aspect of UCA preparation is the quality control assessment (Fig. 11.3). This is even more acute in the case of these rather complex UCA-drug constructs. As for regular UCA, size can be determined by electrical zone sensing, using the so-called Coulter Counter. This is a fast and accurate method to measure at the same time UCA concentration, gas volume, size distribution (in volume and number) and UCA surface. Other methods exist, but so far they have proven less suitable than electrical zone sensing due to technical limitation from the natural buoyancy behavior of UCA in liquid.
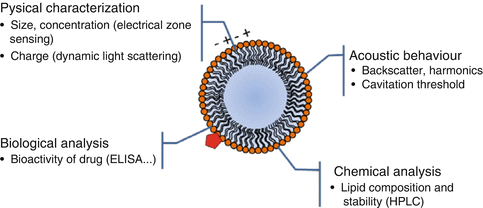
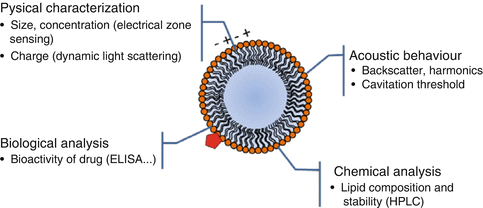
Fig. 11.3
Methods available for the quality control assessment of UCA (Red pentagon represents a drug molecule)
Zeta potential is also an important parameter to assess as it provides information on UCA surface charge. It relies on electrophoretic light scattering technology. The magnitude of the zeta potential gives an indication of the potential stability of the formed UCA. If all the UCA particles in suspension have a large negative or positive zeta potential, then they will tend to repel each other. On the other hand, if UCA particles display low zeta potential values, there will be a tendency for the particles to come together, promoting aggregation behavior. This measure could also be useful to monitor adsorption of nucleic acid molecules on positively charged UCA.
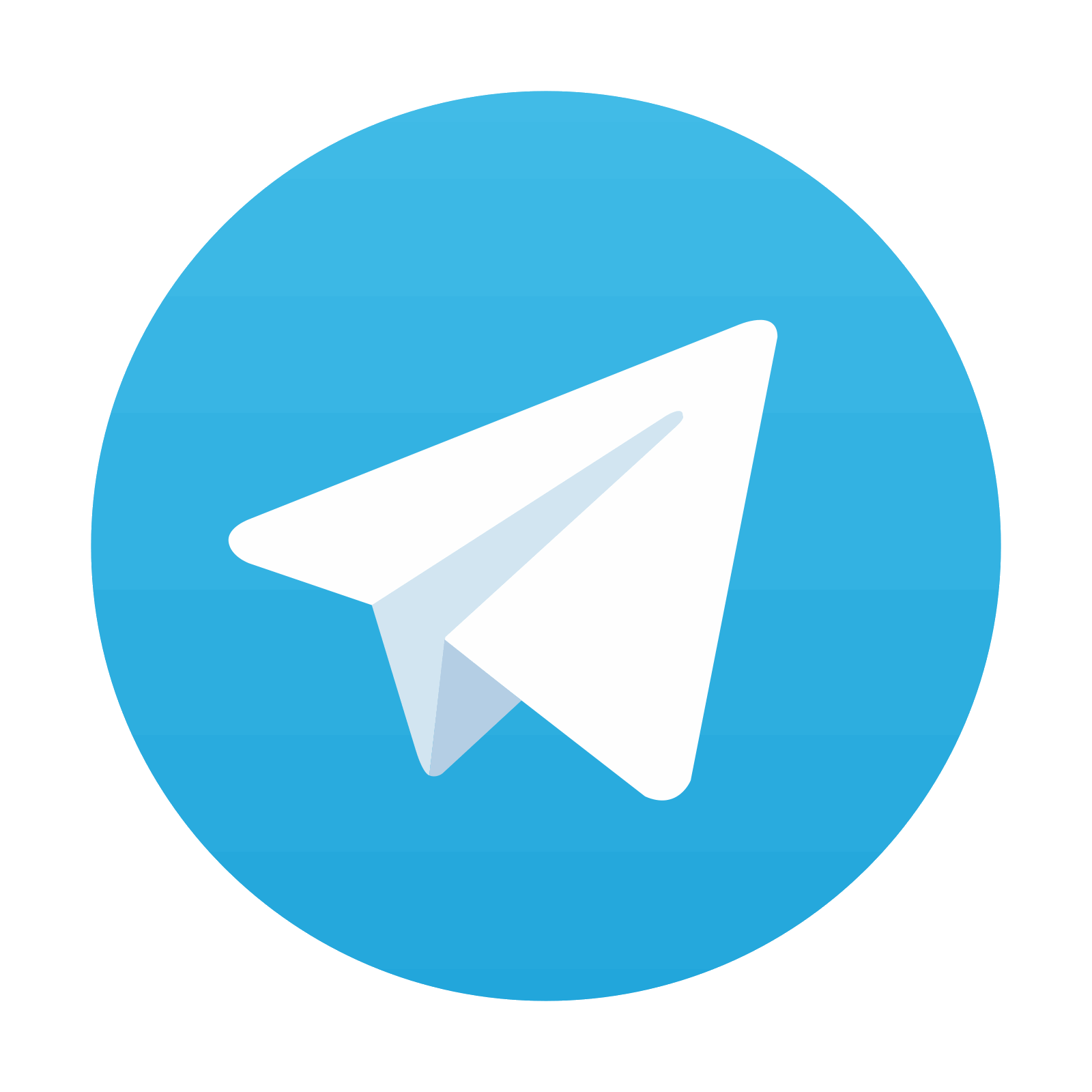
Stay updated, free articles. Join our Telegram channel
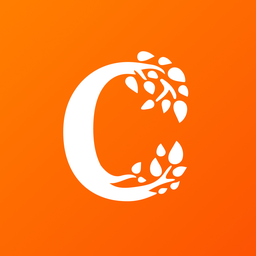
Full access? Get Clinical Tree
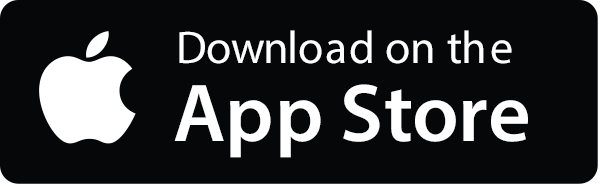
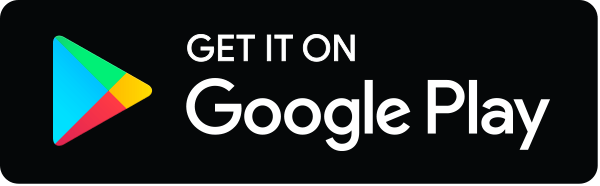