TNM staging system for prostate cancer
Primary tumor(T)
TX
Primary tumor cannot be assessed
T0
No evidence of primary tumor
T1
Clinically inapparent tumor neither palpable nor visible by imaging
T1a
Tumor incidental histologic finding in ≤5 % of tissue resected
T1b
Tumor incidental histologic finding in >5 % of tissue resected
T1c
Tumor identified by needle biopsy (e.g., because of elevated PSA)
T2
Tumor confined within prostate
T2a
Tumor involves one lobe
T2b
Tumor involves more than one half of a lobe but not both lobes
T2c
Tumor involves both lobes
T3
Tumor extends through the prostate capsule
T3a
Extracapsular extension
T3b
Tumor invades seminal vesicle(s)
T4
Tumor is fixed or invades adjacent structures other than seminal vesicles
T4a
Tumor invades bladder neck, external sphincter, or rectum
T4b
Tumor invades levator muscles or is fixed to pelvic wall, or both
Regional lymph nodes (N)
NX
Regional lymph nodes cannot be assessed
N0
No regional node metastasis
N1
Metastasis in single lymph node, ≤2 cm
N2
Metastasis in a single node, >2 cm but not >5 cm
N3
Metastasis in a node >5 cm
Distant metastasis (M)
MX
Presence of metastasis cannot be assessed
M0
No distant metastasis
M1
Distant metastasis
M1a
Nonregional lymph node(s)
M1b
Metastasis in bone(s)
M1c
Metastasis in other sites
Table 19.2
American Joint Committee on Cancer 2010
Anatomic stage prognostic groups | |||||
---|---|---|---|---|---|
Group | T stage | N stage | M stage | PSA | Gleason |
I | T1c | N0 | M0 | <10 | ≤6 |
T2a | N0 | M0 | <10 | ≤6 | |
T1–2a | N0 | M0 | X | X | |
IIA | T1a–c | N0 | M0 | <20 | 7 |
T1a–c | N0 | M0 | ≥10, <20 | ≤6 | |
T2a | N0 | M0 | ≥10, <20 | ≤6 | |
T2b | N0 | M0 | <20 | ≤7 | |
T2b | N0 | M0 | X | X | |
IIB | T2c | N0 | M0 | Any | Any |
T1–2 | N0 | M0 | ≥20 | Any | |
T1–2 | N0 | M0 | Any | ≥8 | |
III | T3a–b | N0 | M0 | Any | Any |
IV | T4 | N0 | M0 | Any | Any |
Any T | N1 | M0 | Any | Any | |
Any T | Any N | M1 | Any | Any |
19.3 Treatment Options
The most established treatment options for favorable to intermediate-risk prostate cancer include radical prostatectomy, external beam radiotherapy, or brachytherapy. There are no published contemporary phase III randomized trials that have directly compared these treatment options to determine which therapy, if any, is superior with regard to outcome and toxicity, and such comparisons may not be feasible [2].
19.4 Historical Review of RT Treatment Options and Outcomes
Conventional 2D RT was initially used to treat early stage prostate cancer, but efficacy at ≤70 Gy was found to be less than previously thought when PSA became available for assessing duration of response [3]. When using 2D techniques to achieve doses >70 Gy, the genitourinary and gastrointestinal side effects increase considerably [4]. However, several retrospective analyses indicated that the delivery of doses higher than 70 Gy improved local control of the tumor [5–7]. Further technological innovations introduced CT-based 3D conformal radiotherapy (3D CRT) delivery systems. These 3D systems enabled radiation oncologists to more safely escalate radiotherapy to doses >70 Gy before reaching the upper limit of acceptable bladder and rectal toxicities [8]. An early study utilizing 3D CRT for the prostate boost portion of treatment demonstrated on DVH analysis that the volume of bladder and rectum receiving the prescribed dose could be reduced to nearly one half of the volume treated with 2D RT delivery [9]. Eade et al. described the dose response for over 1,500 men treated with 3D conformal or IMRT at Fox Chase Cancer Center from 1998 to 2002, demonstrating that there are continued gains of approximately 2.2 % freedom from biochemical failure (FFBF) for every 1 Gy increase, even beyond 80 Gy using either the nadir + 2 or ASTRO definitions of biochemical failure [10].
Several randomized, controlled trials also compared higher dose radiotherapy (>70 Gy) to conventionally dosed radiotherapy (70 Gy) with respect to outcomes in men with prostate cancer [11–15]. In a randomized dose escalation trial of 301 patients, Kuban et al. found an improvement in freedom from biochemical or clinical failure at a median follow-up of 8.7 years of 78 % in the group receiving 78 Gy compared to 59 % in patients receiving 70 Gy [11]. However, gastrointestinal toxicity of grade 2 or greater occurred in 26 % of patients in the high-dose 78 Gy arm compared to 13 % in the conventional 70 Gy arm in treatment delivered by 3D CRT. A meta-analysis that included 7 trials found a significant FFBF benefit for dose escalation when all of the trials (p < 0.0001) were considered [16]. This benefit was significant for NCCN low-risk (p = 0.007), intermediate-risk (p < 0.0001), and high-risk (p < 0.0001) groups, although no difference was found for overall survival (p = 0.69) or disease-specific survival (p = 0.41). All of the trials had less than 10 years of follow-up, which is not sufficient for survival endpoints.
19.5 Clinical Evidence for IMRT
While the 3D CRT prostate cancer dose escalation trials were accruing, intensity-modulated radiotherapy (IMRT) was introduced, revolutionizing the radiation treatment of numerous tumor types, including prostate cancer. In 1996, Ling et al. published one of the first descriptions of prostate cancer inversely planned intensity-modulated photon beams using dynamic multi-leaf collimation [17]. The ability to collimate the treatment fields in a dynamic way during the delivery of radiation resulted in more conformal dose distributions with the potential to reduce toxicity.
When compared directly to 3D CRT plans in dosimetric studies, IMRT proved superior in target volume coverage at the prescribed dose and at reducing the volume of normal tissues treated to specified constraint doses [18–22]. Ailleres reported that 95 % of the PTV1 received 5 Gy more with IMRT when compared to 3D CRT planning without compromising dose limits on the bladder and rectal walls [23]. Using an endorectal balloon for prostate immobilization, Ashman compared sequential IMRT plans to 3D CRT in delivering whole pelvic radiotherapy and found that IMRT reduced the volume of bowel receiving 45 Gy by 60 % when compared to 3D CRT delivery [21]. Without placing intentional constraints on the penile bulb dose, Kao designed IMRT and 3D CRT plans to deliver 74 Gy to the target for ten patients with clinically organ-confined prostate cancer; IMRT reduced the mean penile bulb dose, the percentage of bulb receiving >40 Gy, and the dose received by >95 % of the penile bulb; however, the maximum penile bulb dose was higher to a very small volume [22]. Subsequent studies have confirmed the reduction in dose to critical structures including the penile bulb with IMRT while achieving improved coverage to target structures including the pelvic lymph nodes [24, 25].
Investigators at Memorial Sloan Kettering Cancer Center were leaders in the application of IMRT to prostate cancer. Zelefsky et al. [26] described the early experience where 61 men with localized disease were treated with 3D CRT and 171 with IMRT to a prescribed dose of 81 Gy. When comparing the plans of 20 randomly selected patients, IMRT showed a significant improvement in the coverage of the clinical target volume (CTV) to the prescribed dose while lowering the volume of the bladder and rectal walls that received this dose. The analysis also demonstrated a reduction in the 2-year risk of rectal bleeding from 10 % with 3D CRT to 2 % for IMRT [26]. In a larger analysis, Zelefsky analyzed 1,100 patients with cT1c–T3N0 prostate cancer treated with 3D CRT or IMRT and reported statistically significant improvements in the 5-year PSA relapse-free survival in the favorable, intermediate, and unfavorable risk groups. For the patients who received a prescription dose of 81 Gy, IMRT resulted in significantly less late grade 2 rectal toxicity (14 % vs. 2 %) with no impact on GU toxicity [27]. In the Dutch randomized dose escalation trial CKVO 96-10, a subset analysis comparing the toxicities for patients who received 78 Gy via sequential 3D CRT or via simultaneous integrated boost, with IMRT, IMRT resulted in significantly lower acute grade 2+ GI toxicity (61 % vs. 20 %), while the rates of 5-year FFBF were comparable in both groups (61 % vs. 70 %). Acute GU and late GI and GU toxicities were similar in both groups [28]. Several other reports confirmed that IMRT is a safer delivery method for high-dose RT to the prostate [18, 23, 29], which eventually resulted in the adoption of IMRT as the standard of care.
IMRT is associated with an increased volume of low-dose radiation to normal tissues as a result of the multiple gantry angles by which IMRT is delivered to create the highly conformal dose distribution to target structures. Kry reported that IMRT required 3.5–4.9 times more monitor units (MU) when compared to more conventional treatments. According to the analysis, the calculated conservative maximal lifetime risk of fatal radiation-induced malignancy was 1.7 % for conventional RT, 2.1 % for IMRT with 10-MV X-rays, and 5.1 % for IMRT using 18-MV X-rays [30]. There are conflicting data on the observed risk of second malignancies after prostate cancer radiotherapy [31–35], and the overwhelming majority of clinical and dosimetric evidence support IMRT as the standard of care over 3D CRT.
19.6 IMRT Technique
Close collaboration between physicians, nurses, radiation therapists, dosimetrists, and medical physicists facilitates coordination that can reduce uncertainties in IMRT treatment delivery. In our institution, prior to diagnostic/planning MRI and CT simulation, the patient receives instructions on bowel and bladder preparation. Table 19.3 outlines the simulation technique and sequence that is utilized at the University of Miami.
Table 19.3
Simulation technique and sequence
1. Diagnostic MRI. A 3.0 T MRI using a pelvic body coil is acquired when possible with the following sequences: T2, T1 plain, and T1 with dynamic contrast enhancement (DCE) and diffusion-weighted imaging (DWI) in 2–2.5 mm slices throughout the pelvis. This is a diagnostic staging exam that is fused to the planning CT. The same bowel preparation is recommended for the MRI and CT simulation. |
(a) Bowel prep: the patient is encouraged to abstain from drinking carbonated beverages and gas-producing foods starting the day before imaging. The evening before, magnesium citrate is consumed to empty the bowel. An enema or two are administered within 2 h of imaging. |
2. Placement of four gold fiducial markers (tracking beacons or gold seed fiducials) transrectally. |
3. Wait a minimum of 7 days for fiducial marker position to stabilize. |
4. On our clinical trials, we have also performed a second limited 2–2.5 mm slice thickness MRI simulation using T2w, T2*, and DWI sequences. An MRI after fiducial marker placement aids in aligning the MRI and CT simulation images. This is not usually reimbursed when attempted outside of a clinical trial, but is very helpful in image fusion. |
5. CT simulation at 2.0–2.5 mm slices throughout the pelvis in supine position with legs in an immobilization device. |
6. CT-MR fusion. |
All patients who are candidates for an MRI with contrast undergo diagnostic 3.0 T MRI of the prostate and pelvis using a body coil. Multiparametric MRI that includes T2w, T1 non-contrast, T1 dynamic contrast-enhanced (DCE)-MRI, and diffusion-weighted imaging (DWI) sequences (our standard sequences), as well as MR proton spectroscopy (MRS), has been shown to improve the sensitivity and specificity of tumor localization [36–39]. T2w-MRI provides an excellent depiction of prostate and pelvic anatomy with regions of healthy peripheral zone prostate tissue demonstrating higher signal intensity than prostate cancer. The observed reduction in MRI image signal intensity is due to a loss of the normal glandular (ductal) morphology in regions of prostate cancer. However, other benign pathologies such as inflammation, benign prostatic hyperplasia (BPH), blood, and prior radiation treatment also cause a loss of ductal morphology and low signal intensity on T2w-MRI. Additionally, infiltrating prostate cancer does not always cause a reduction in normal glandular morphology and therefore may not be hypointense on T2w-MRI. Due to these confounding factors, T2w-MRI alone can localize cancer larger than 0.5 cm3 in volume with only a 65–74 % sensitivity and low specificity [40]. Other studies also report quite variable sensitivity (50–83 %) and specificity (21–88 %). Utilizing an endorectal coil improves MRI’s sensitivity to 78 %, but the specificity still remains poor (55 %).
MRS, DWI, and DCE-MRI have been used to improve the sensitivity and specificity of MRI and to determine tumor location and extent. Localization accuracies of above 80 % may be achieved by combining these methods [41]. Each has shown promise when combined with T2w-MRI. DWI is sensitive to random thermal movement of water molecules and provides a determination of stiffness. DWI is used to calculate apparent diffusion coefficient (ADC) values, which are significantly lower in tumor than in normal prostate due to the restriction of water displacement. Lower ADC values are associated with Gleason 7 or above disease [42].
DCE-MRI also discriminates between normal and cancerous tissues [43, 44]. Greater and earlier enhancement with washout over time is seen in tumor tissue vs. delayed enhancement in normal tissue. DCE-MRI is a measure of tissue vascularity and angiogenesis. Specificity has been notably high at close to or over 90 % in a number of studies [45, 46], while sensitivity has been 65 to >80 %. At the University of Miami, we routinely use DCE-MRI combined with DWI (and ADC maps) and T2w-MRI in our assessments and planning of radiotherapy. These sequences aid in the determination of tumor location and extraprostatic extension, thereby informing the CTV. In addition, there is an emerging literature on delivering higher daily doses using dose painting to the dominant prostatic lesion(s) based on MRI criteria [47–54], and we are testing this approach in clinical trials (Fig. 19.1). In some high-risk patients with well-defined MRI lesions, we have boosted the dominant intraprostatic lesion(s) slightly (2.15 Gy per fraction to 86 Gy) if not a candidate for a clinical trial.
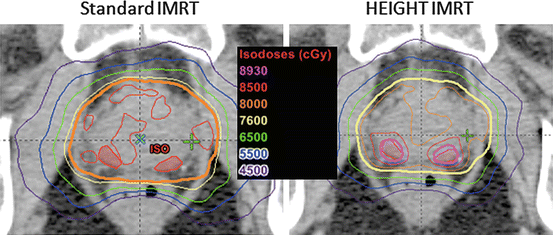
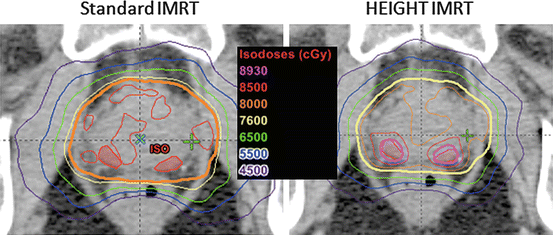
Fig. 19.1
Dose distribution on a single axial slice for a dose-painting IMRT plan prescribing a boost to dominant intraprostatic lesion(s) on multiparametric MRI for patient on an inhouse (UM) clinical trial
Patients are given bowel preparation and bladder filling instructions for the diagnostic MRI to mimic treatment bladder and rectal filling. If possible, the MRI is performed on a flat tabletop to most accurately reproduce the patient’s treatment position. After the MRI, 3–4 gold fiducial markers are implanted transrectally in the prostate at a distance of 1 cm apart [55], to reduce interfraction setup error [56–59]. Seed migration is not a major issue [57, 58, 60, 61]. We typically wait a week after fiducial marker placement before simulation to allow for the stabilization of fiducial position.
Gold seed fiducials are currently the most commonly employed method to correct for interfraction motion. Although patients do require an extra outpatient procedure, they tolerate the insertion well [62]. The most frequent reported side effects are hematuria (15 %) and rectal bleeding (4 %) [63]. Patients report their pain is less than that of diagnostic biopsy, even without local anesthesia. Daily fiducial marker alignment prior to each treatment via orthogonal portal imaging, cone beam CT, or in-room CT reduces uncertainties from interfraction motion [56], allowing for reduced margins [58, 59, 64, 65].
Daily alignment to gold seed fiducials reduces the subjectivity of the alignment process over cone beam CT (CBCT) alone. With fiducial correction for interfraction motion and arc-based therapy that typically takes <5 min (2–3 arcs), we use a PTV expansion of 5 mm everywhere, except posteriorly where the margin is 3–4 mm. Langen et al. have shown that the chance for prostate displacement increases over time during patient setup [66]; arc-based therapy reduces the chance of a portion of the CTV falling outside the PTV.
Real-time tracking of fiducials using fluoroscopy, electromagnetic transponders, or transperineal ultrasound [67] limits effects from intrafraction motion, which takes on considerable importance. When using transponders, three are placed in the prostate to define prostate position [68]. The system continuously monitors and compares the triangulated center of mass of the transponders to the planned isocenter. This information is used to stop the treatment if a predetermined threshold is exceeded [69]. The threshold that has been used in our institution is 3 mm. Of note, transponders result in significant artifacts on MRI that preclude assessments of tumor location or recurrence in the prostate.
After waiting 7 days to allow for fiducial marker position to stabilize, CT simulation with 2 mm slices is performed throughout the pelvis in supine position with arms on chest and legs supported or in an immobilization device such as a Vac-LokTM. On the same day, a limited 2.5 mm slice thickness MRI simulation using T1, DWI, T2*, and T2w sequences has been used for patients on protocols in which a GTV boost is administered in order to facilitate more accurate MRI-CT fusion based on the position of the implanted markers. While the simulation MRI is of value in fusing the diagnostic MRI to the planning CT, it is not usually reimbursed.
Bladder and rectal volumes should be thoroughly analyzed prior to approval of the CT simulation for IMRT planning. At our institution, we have a low threshold for repeating the CT simulation after the patient has adjusted the bladder filling or rectal emptying if these normal organs do not conform to our preferred volume and anatomic orientation. The smaller the rectal volume at simulation, the more the plan will reflect a worst case dosimetric assessment during planning.
19.7 Contouring of Target and Normal Anatomy
The IMRT planning process begins with the fusion of the CT and MRI images followed by delineation of the target structures and normal tissue volumes by the treating radiation oncologist. As background, the prostate is an exocrine gland that surrounds the prostatic urethra. The normal adult gland measures approximately 4×3×3 cm (transverse, AP, craniocaudal) and weighs 15–20 g [70]. The size of the prostate generally increases with age, and according to Zackrisson et al., up to 85 % of healthy males older than 40 years have prostate volumes higher than 20 cm3 [71]. Five anatomical prostatic zones are recognized: (1) anterior fibromuscular stroma, (2) periurethral glandular tissue, (3) transition zone, (4) central zone, and (5) peripheral zone [70].
Inconsistencies in CT contouring are the result of poor definition of the prostate relative to adjacent structures and wide variation in anatomic position relative to the pelvic bones [72]. The prostate tends to blend in with muscle, making it unclear where the prostate borders are located relative to the levator ani muscle, the rectum (particularly inferiorly near the prostatic apex), and the bladder wall superiorly. The inferior aspect of the prostatic apex is not recognizable on CT. The rectum blends into the apex posteriorly, and the urogenital diaphragm blends into the apex inferiorly. These structures are more clearly visualized on T2w-MRI [73]. Using CT alone, the apex location may be estimated to be about 1.0–1.5 cm superior to the bulb of the penis. It is best to overestimate the inferior location of the apex in the absence of MRI. MRI considerably resolves these boundaries and makes accurate contouring of the prostate more consistent [74, 75]. MRI prostate volumes are more aligned with ultrasound volumes [76]; CT prostate volumes are about 30–40 % larger than MRI volumes [77, 78]. However, current planning algorithms are based on CT, making CT-MR fusion the best approach to define the prostate, seminal vesicles, and pelvic lymph node regions.
CT-MR fusion is fraught with potential problems that could lead to significant errors if not performed appropriately. The CT should be used primarily and the MRI only used as a reference because of the inherent problems with the accuracy of CT-MR fusion. The position of the prostate on MRI may be substantially different from the CT because of bladder and rectal filling. These differences are accentuated when the MRI is being performed at a different time (not in sequence with the CT) and on a concave tabletop (instead of a flat table on the CT simulator). Patients should be given instructions on diet to minimize gas and should perform an enema before going for both the MRI and CT. Ideally, a radiation simulation therapist will be present at the MRI to confirm optimal bladder and bowel filling at the time of MRI. An MRI equipped with lasers to ensure accuracy of patient positioning will also help to optimize the subsequent CT-MR fusion. If there are considerable differences in bladder and rectal filling, the fusion will be inaccurate. The random error for CT-MR registration along the three spatial directions was estimated to be on the order of 0.5 mm and around 0.4° in rotation (standard deviation) for each axis [79]. Fusion error is minimized when a second limited MRI simulation (T1, DWI, T2, and T2* MRI sequences) with fiducials in place on the same day of CT simulation is performed and the fiducial markers are considered in the fusion process [54].
The seminal vesicles (SV) are paired organs located in the connective tissue lodged between the urinary bladder and the rectum lateral to the ampulla of the vas deferens. Seminal vesicles can vary in size, and differences in dimension between the right and left seminal vesicle have been reported [80, 81]. According to surgical specimen reports, the length is about 31 ± 10.3 mm [80], which is concordant with reported results on ultrasound [71]. The angle between the seminal vesicles and the horizontal plane (normally 50 to 60°) changes with bladder and rectal filling [82]. Seminal vesicle contouring, similar to that of prostate contouring, is better delineated on MRI because of enhanced anatomic detail [83]. We include the proximal SVs in the CTV with the prostate.
The bulb of the penis is formed by the elongation of the corpus spongiosum after the separation of the corpora cavernosa to form the crura of the penis. The bulb of the penis is attached superiorly to the inferior surface of the urogenital diaphragm [84]. The penile bulb is best visualized on T2w-MRI as an oval-shaped, hyperintense midline structure [25, 84]. Although the penile bulb can also be identified on CT imaging and transverse transrectal ultrasound, MRI is best for the superior and inferior aspects. Contouring should stop inferiorly when the bulb loses the lateral bulging aspects of the corpus spongiosum. As summarized by Van der Wielen et al. [85], the sparing of the penile bulb, corporal bodies, and neurovascular structures has sometimes been associated with increased preservation of erectile function, but results have been mixed [25, 86]. IMRT reduces the dose received by the penile bulb, as shown by some authors and reported above [22, 87]. Regarding the relationship between the penile bulb dose and the development of erectile dysfunction, some studies have not shown any significant association [88], whereas Merrick et al. have [89]. Roach et al. advocate keeping the penile bulb dose to <52.5 Gy [90].
19.8 IMRT Planning and Dosimetry
Table 19.4 outlines common dose constraints utilized in IMRT planning for prostate cancer. Figure 19.2 demonstrates a typical IMRT treatment plan with corresponding dose-volume histograms when prescribing 80 Gy in 40 fractions to the PTV, the standard dose used at the University of Miami. At the University of Miami, particular attention is paid to the 30 Gy isodose line, and optimization structures are created to ensure that this line is anterior to the posterior rectal wall, as well as excluding the lateral rectal wall as much as possible (Fig. 19.3). The bladder volume at 30 Gy is also reduced by designing a plan that pulls in dose tightly in the anterior. The resultant optimization of dose across the rectal and bladder is accomplished using optimization structures that result in an increase in doses to the lateral soft tissues and femoral heads while still maintaining constraints to the femoral heads (Fig. 19.4).
Table 19.4
Common dose constraints for prostate IMRT
Dose (Gy) or dose-volume parameters | |||
---|---|---|---|
Organ | UM: 80 Gy/40 fx | RTOG 0126 arm 2: 79.2 Gy/44 fx | MSKCC: 86.4 Gy/48 fx |
PTV | V80 Gy ≥95 % | V79.2 ≥ 98 % | V86.4 ≥ 95 % |
V92 Gy(115 %) = 0 % | V84.7 ≤ 2 % | V95.04 = 0 %
![]() Stay updated, free articles. Join our Telegram channel![]() Full access? Get Clinical Tree![]() ![]() ![]() |