Fig. 22.1
Axial CT images of adult and pediatric patient treated with IMRT. Images are at the same scale (orange bar). Since the physical characteristics of the delivery hardware are independent of the patient anatomy, adults benefit from larger distances between targets and tissues at risk. In the upper right corner, there is a representative 5 cm dose falloff for an IMRT plan (6 MV flattening filter free, arc delivery, 2.5 mm MLC)
For treatment delivery, IMRT requires rigid immobilization devices which may include anesthesia and daily confirmation of setup using image-guided radiation therapy (IGRT) to ensure the spatial accuracy of the treatment (Fig. 22.2). The additional procedures added to the already prolonged treatment times for static IMRT led investigators to query whether IMRT was practical in pediatric and young adult patients. Furthermore, motion compensation techniques such as breath hold and respiratory gating, allowing for beam-on timing to be controlled and correlated to the phase or amplitude of the patients breathing, were not deemed feasible for pediatric cases. However, literature has shown that motion compensation is applicable for pediatric cases, at the expense of longer treatment times [8, 9]. Optimized simulation workflows, improved anesthesia solutions, increased experience with IGRT and gating practices, and optimized IMRT plans with arc delivery technology [10], aided by the introduction of high-dose-rate clinical beams (≥10 Gy/min) [11], have the potential to reduce the end-to-end time in the treatment room for children, while reducing radiation exposure to normal tissues.
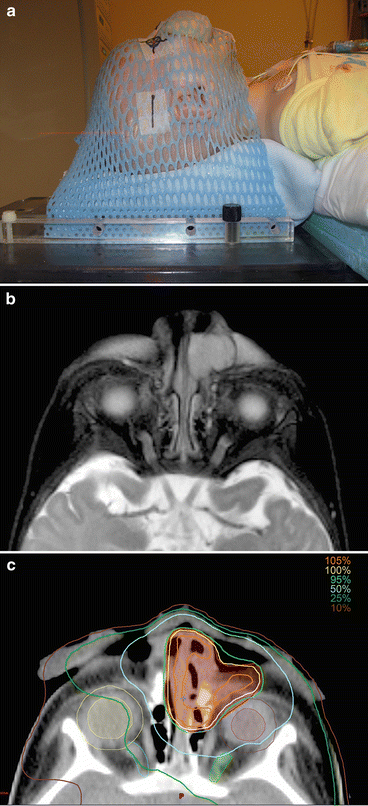
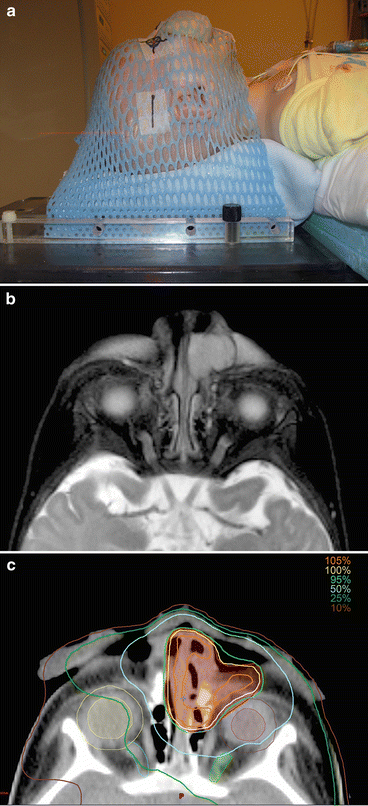
Fig. 22.2
IMRT process for pediatric patient with rhabdomyosarcoma of the nostril. (a) Treatment setup under full anesthesia; thermoplastic mask is used to immobilize the patient and the custom bolus, offering a high degree of positioning reproducibility. (b) Patient pretreatment MRI images are available for fusion to treatment planning CT to accurately delineate the span of the tumor and adjacent normal tissues. (c) Planning CT with dose distribution for VMAT delivery with 6 MV photons (target is delineated in red, with transparent red filling; also present right/left globes and corresponding 3 mm expansions and optic nerves)
The therapeutic advantages of IMRT to conform therapeutic doses to the target volume while avoiding critical organs and normal tissues were rationale enough to study its feasibility in pediatric cases. In 2004, Penagaricano et al. reported their clinical experience treating five pediatric malignancies using IMRT and indicated that the technique was a viable alternative to conventional 3D treatment [12]. In 2006, Bhatnager et al. reported on their IMRT experience while treating 22 pediatric tumors involving different sites. Immediate benefits were observed in significant dose reduction for OARs (6–42 % of the planned target dose) which was demonstrated in the pituitary, brainstem, cochlea, optic nerves, and lens during intracranial irradiation. Spinal cord and parotid dose reductions of up to 50 % of the planned dose were observed in head and neck cases, while for pelvis treatments, the dose to the bladder, rectum, and small bowel was reduced by 22–63 % of the target dose [13]. In 2009, Sterzing et al. reported a single institution IMRT experience in children, with long-term outcomes spanning 9 years, validating that local control was not compromised using IMRT in the pediatric population, and there was a significant reduction in normal tissue exposure in the high-dose radiation volume [14]. Other observed clinical benefits of IMRT include fewer cutaneous and subcutaneous late effects such as pigment changes, hair loss, telangiectasia, and subcutaneous fibrosis. The significant reduction in superficial dose to the cutaneous and subcutaneous regions has been demonstrated in dosimetric studies [15] and attributed to an increased number of incident beams, spreading dose over more skin area which inherently lowers the dose buildup in the superficial tissues.
22.1.1 The Main Challenge: Extraneous Radiation Exposure of Normal Tissues
Extraneous dose is the unavoidable low dose radiation which the patient receives outside the planned target volume. In all radiation treatment modalities, including IMRT, normal tissues receive low dose radiation from head leakage, patient internal scatter, and collimator scatter sources (Fig. 22.3). The main disadvantage of IMRT is that a larger volume of unintended normal tissues are exposed to low dose radiation as a result of higher monitor units with complex multi-field plans and increased treatment times and greater neutron contamination for higher energy treatments [18]. Some authors argue that the extraneous low dose radiation may contribute to a higher rate of secondary malignancy(s). While the estimation of radiation-induced secondary malignancy(s) is an area of active research, radiation biologists have modeled risks for second malignancy(s) in the low dose region to be approximately “8 % per Gy probability for a fatal cancer to develop due to radiation above spontaneous incidence. An estimated 0.75–1 % of surviving patients would be expected to develop a second malignancy as a consequence of IMRT, approximately double the incidence of secondary malignancies due to conventional therapy” [19–22].
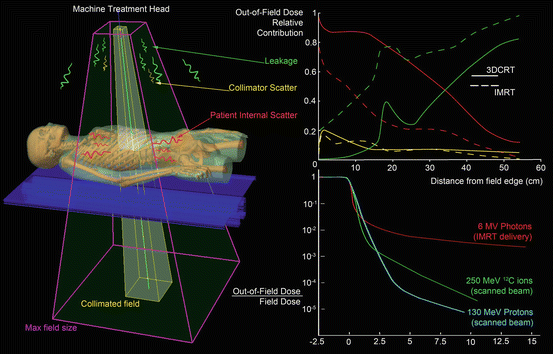
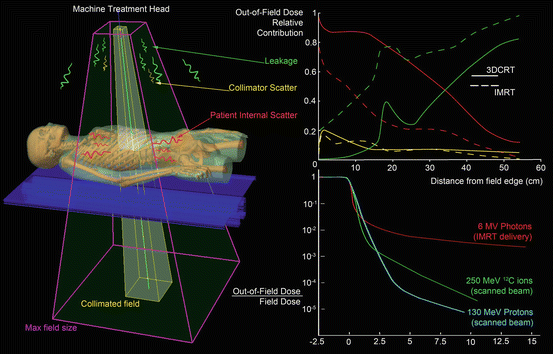
Fig. 22.3
Extraneous or out-of-field dose has three sources (leakage, patient internal scattering, collimator scattering) and is defined as the dose that the patient receives outside the treatment field. Leakage increases for IMRT delivery versus 3DCRT, the opposite is true for patient internal scatter, while collimator scatter contribution is relatively constant as seen in the graph in the upper right corner (Data adapted from Ref. [16]). The out-of-field dose is significantly reduced using charged particles versus photons as indicated in the graph in the lower right corner (Data adapted from Ref. [17])
In addition to second malignancy(s), radiation-induced sequelae to radiosensitive organs outside the radiation field need to be considered. Organs/tissues which may be highly susceptible to extraneous low dose radiation include gonads, breast tissue, thyroid, and lens (infertility or sterility, cessation or abnormal growth, hypothyroidism, cataracts) [23, 24]. Irreversible radiation-induced injury to the gonads can significantly alter quality of life for childhood cancer survivors who desire an offspring later in life. Since the gonads are one of the most radiosensitive organs, even exposure to low dose radiation may inadvertently contribute to infertility or sterility [25]. Thyroid and lens are also very radiosensitive structures which can be affected by very low dose radiation. Breast tissue is highly susceptible to the mutagenic effects of ionizing radiation in young females. The risk for breast cancer in Hodgkin’s lymphoma survivors who receive chest irradiation remains high even for patients treated with conformal techniques such as IMRT. Recent reports show that the majority of female childhood cancer survivors who receive low dose (median 14 Gy) radiation therapy to large volumes (e.g., whole-lung irradiation) have a significantly elevated risk for developing breast cancer [26].
While studies show that differences between IMRT and 3DCRT regarding peripheral extraneous doses received by gonads, thyroid, breast tissue, and lens in children and young adults are minimal [27], they should always be considered, calculated, and/or directly measured for IMRT plans. Dose distributions therefore may influence clinical decisions where pediatric radiation oncologists may consider 3DCRT versus IMRT to protect sensitive structures from the low dose irradiation. However, it is important to know that the overall absolute extra-target peripheral dose for the two modalities is influenced by different “competing” dominant effects (Fig. 22.3). For 3DCRT patient, internal scatter is larger resulting in higher out-of-field doses closer to the target region, while with IMRT, there is an increased head leakage corresponding to higher peripheral doses far from the target region [28]. Therefore, the increased monitor units delivered with IMRT should not be treated as a single, accurate indicator of increased out-of-field peripheral irradiation and subsequent low dose exposure of normal tissues [28].
22.1.2 Strategies to Minimize the Out-of-Field Dose
Given the observed benefits of organ-/tissue-sparing capabilities of IMRT in the high-dose region, several strategies have been developed and demonstrated to minimize the unintended out-of-field dose from IMRT delivery. Using coplanar IMRT beams may be one technique to minimize the internal scatter dose. Kan et al. reported on five cases with different pediatric malignancies in the head and neck planned with both coplanar and noncoplanar IMRT techniques. It was observed that peripheral doses were 1.8–2.5 times higher while using the noncoplanar beams [29]. Another technique to reduce out-of-field dose is the removal of the flattening filter, which removes a neutron contamination source associated with high-energy photons interacting in the filter, reduces head leakage, and reduces the delivery time. Cashmore et al. tested this hypothesis with a linear accelerator outfitted with conventional (flattened) and flattening-filter-free modes. IMRT treatment plans for pediatric intracranial treatments were delivered to phantoms using both approaches. Measurements indicated a 23–70 % reduction in peripheral doses (from thyroid to gonads) using the flattening-filter-free modes [30].
The reduction in beam-on time (number of monitor units) is also a method to minimize out-of-field peripheral doses. Besides significantly increasing target coverage and reducing the delivery time, VMAT delivery of IMRT plans has been shown to generally match or even reduce the treatment monitor units compared to conventional static field IMRT plans [31]. In complex pediatric pelvic cases, VMAT reduced the treatment time by 78 % and monitor units by 25 % compared with standard IMRT as reported by Matuszak et al. [32]. The reduction in out-of-field peripheral dose associated with the delivery and reduction in monitor units was as high as threefold for thyroid during pelvic irradiation [31]. There is a variety of commercially available treatment planning systems, each offering optimization solutions that can be employed in reducing extraneous dose in pediatric patients. Attalla et al. reviewed current planning systems offering IMRT optimization. In this study, IMRT plans using step-and-shoot were designed for pediatric head and neck and CNS cases necessitating simultaneous integrated boosts. The authors observed that while three different commercial treatment planning systems were used to obtain optimized IMRT plans achieving the same clinical objectives, using the same energy, number, and direction of beams, the resulting plan quality was not comparable. They found one system was superior producing more efficient plans, fewer segments and MUs, shorter treatment delivery times, and better conformality [33]. Dose painting (DP), simultaneous integrated boost (SIB), and simultaneous modulated accelerated radiotherapy (SMART) are planning techniques which allow highly customized, highly conformal dose distributions while treating multiple targets to different dose levels and sparing more normal tissue [34, 35].
Due to its complex resulting spatial distributions of dose deposition and high gradients between targets and adjacent normal tissues, IGRT is necessary when using IMRT. Even with anesthesia minimizing the risk for movement during treatment, the precision in executing the treatment plan relies on accurate alignment with daily in-room verification using kV imaging. The use of three-dimensional alignment using cone beam computed tomography (CBCT) adds additional accuracy of setup alignment, particularly in the head and neck region. Additionally, it aids in the decision as to whether replanning is necessary for tumors regressing during the course of treatment or for anatomical changes during the course of radiation such as rapid weight loss. The benefit from frequent CBCT portal imaging must be carefully weighed against the risk for exposure to nontherapeutic ionizing radiation. Daily IGRT imaging doses should be recorded for the cumulative exposure above the prescribed therapeutic dose [36]. The dose contribution from imaging is generally less than 2–3 % of the prescribed dose and is usually neglected from total dose summations [37]. However, for pediatric patients, sensitive structures such as the testes or ovaries may be affected if daily kV planar imaging or CBCT was to be used. For example, testicular doses from kV imaging can be 3–4 times higher than the actual incidental dose from pelvic irradiation treatments. Reducing the imaging field of view to exclude the testes may significantly decrease dose to this region [38, 39].
22.1.3 Future Directions
While refinements in delivery methods, increasing positional accuracy, and decreasing delivery time are critical in minimizing extraneous radiation dose exposure in pediatric patients, the physics of photons’ interaction with tissue does not allow for complete avoidance of normal tissue exposure to low dose radiation. The pediatric oncology community has been investigating intensity modulated proton therapy (IMPT) as the step beyond IMRT in radiotherapy [40]. Many proponents of proton therapy to treat childhood cancer refer to the desirable beam characteristics which minimizes the out-of-field dose (see Fig. 22.3). Protons, unlike photons, deposit most of their energy at the distal end of their range (Bragg peak). This implies practically nonexistent exit dose, while the entrance dose is lower compared to photon attenuation in tissue [41].
There are two main delivery methods in proton therapy: passive scattering and spot scanning. Passive scattering employs beam scatterers to spread out the input beam laterally, metal apertures to collimate the beam, and range modifying devices to spread out the Bragg peak. In principle, passive scattering employs both beam energy and intensity modulation. However, IMPT refers to the second delivery method. Spot scanning, also referred to as pencil beam scanning or modulated scanning, delivers the treatment layer by layer in raster format scanning a pencil beam using powerful magnets. Depth is changed by switching energy, hence changing the range of the protons. Modern delivery systems are increasingly efficient, allowing for very quick delivery of dose even for large target volumes [41].
The primary advantage that high-energy proton therapy has over photon therapy is reducing normal tissue dose [41]. Several studies have modeled the relative risk associated with normal tissue irradiation for both proton treatments and current state-of-the-art IMRT. Athar et al. reported that the potential organ-specific second cancer lifetime attributable risks would be from unintended internal scatter or leakage out-of-field low dose irradiation from 6 MV IMRT and passive scattering proton therapy. The modeling study included data from patients ranging in age from 9 months to 14 years old and one adult and two treatment sites (brain and mid-spine). The lifetime attributable risk for developing a thyroid cancer after treatment to a brain lesion in a 4-year-old patient was estimated at 1.1 % for IMRT versus 0.3 % for proton therapy. The lifetime attributable risk for developing a bladder cancer after treatment to a mid-spine field was estimated to be 0.2 % with IMRT and 0.02 % with proton therapy, suggesting a distinct advantage to proton beam especially with regard to organs at risk further away from the field [42]. Lifetime attributable risks were also modeled to assess the risk for developing radiation-induced tumors within the path of the beam. Using whole-body phantoms for a 4- and 14-year-old child, plans were generated for optic glioma and vertebral body Ewing’s sarcoma. The lifetime attributable risks were modeled for these cases, and the results showed that the risks associated with proton therapy were lower by a factor of 2–10 in the case of protons [43]. In both cases, long-term follow-up is needed to confirm the premise that proton beam therapy is associated with fewer radiation-induced secondary malignancy(s) [44].
Greco et al. reviewed the current trends in proton therapy for children and concluded that IMPT has the potential to “yield superior dose distributions to photon IMRT, with the added advantage of a significant reduction in the volume of healthy normal tissues exposed to low-to-medium doses” [45]. Lomax et al. emphasized the versatility of scanning beam IMPT, where the individual Bragg peaks can be delivered from any field and can be distributed in 3D throughout the target volume, providing an increasing amount of degrees of freedom for designing dose distributions when compared to IMRT or conventional proton therapy [46].
There are problems to be solved with IMPT. The protons relative biological effectiveness (RBE) is spatially variable across the energy deposition curve and maximized at the Bragg peak [47]. Furthermore, the uncertainty in the range of protons in tissue is affected by imaging, patient setup, beam delivery, and dose calculation techniques [48]. High-energy charged particles such as protons yield a significant neutron spectrum in their interaction with the beam line components and the patients’ body, increasing the out-of-field dose [49, 50]. A major hurdle to overcome is that proton facilities are very costly to build and operate, limiting the feasibility of using this modality in many places around the world.
22.2 IMRT Clinical Applications by Tumor Type
22.2.1 Central Nervous System Tumors
22.2.1.1 Medulloblastoma
Medulloblastoma is the most common malignant childhood brain tumor, representing 15–20 % of all central nervous system (CNS) tumors, with the highest incidence in children between 4 and 7 years of age. The cerebellum is the most common site of origin, and there is a distinct propensity for cerebrospinal fluid (CSF) dissemination. Metastatic disease at diagnosis occurs in approximately 30 % of patients; however, with aggressive therapy, two-thirds of the patients are long-term survivors [51].
Craniospinal irradiation (CSI) with a boost to the primary site has long been part of the multimodality management, which includes surgery and chemotherapy. Conventional CSI therapy for standard risk medulloblastoma patients consists of 3DCRT with two opposed lateral whole-brain fields matched to a posterior spine field to comprehensively cover the CSF with doses as high as 36 Gy, followed by a selective boost to treat the entire posterior fossa (if primary site) using doses as high as 54 Gy [52]. This treatment is associated with severe significant late effects including trunk shortening (spine), severe ototoxicity (cochlea), xerostomia (parotid), permanent hair loss (scalp), endocrine (hypothalamic-pituitary axis), and neurocognitive effects (temporal lobes/hippocampus) [53]. Children’s Oncology Group (COG) protocols are testing the safety of reducing CSI doses and limiting boost target volumes to the tumor bed plus a circumscribed margin. Further refinement of the boost volumes using natural barriers to tumor spread such as bony calvarium or tentorium can further reduce boost target volumes. The optimal PTV volumes and doses for CSI and primary site boost for medulloblastoma have yet to be validated in prospective clinical trials.
Incorporating IMRT in CSI treatments results in higher conformality, dose homogeneity, and normal tissue sparing – primarily in the cochleae, temporal lobe and hypothalamic-pituitary tract but also organs in the beam pathway targeting the spinal CSF (the thyroid, heart, lung, esophagus, vertebral bodies, esophagus, stomach, kidneys, liver, small bowel, and gonads). The feasibility of CSI-IMRT has been confirmed by several investigators [54–58]. CSI-IMRT allows for a dosimetric match at the junction of cranial and spinal fields and increased dose homogeneity across the matched fields when compared to 3DCRT. Furthermore, there is significant reduction in exposure to normal tissues while providing superior coverage of the craniospinal axis (Fig. 22.4). Further reduction in normal tissue exposure is reported with removing flattening filters [59]. Enhanced reliability of setup with kV image guidance and using quicker delivery techniques such as VMAT delivery with high-dose rate beams reduce beam-on time while allowing for greater reliability of delivering the prescribed radiation dose [54, 60].
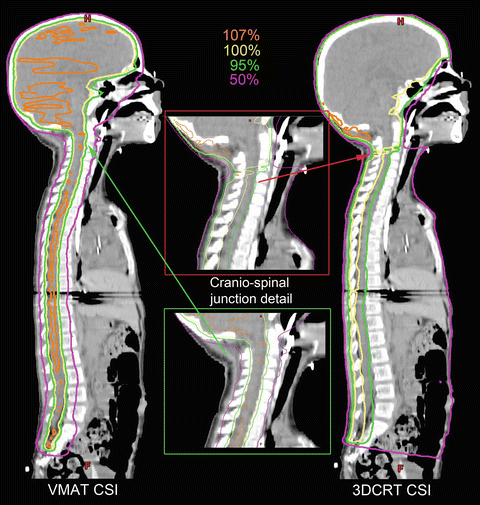
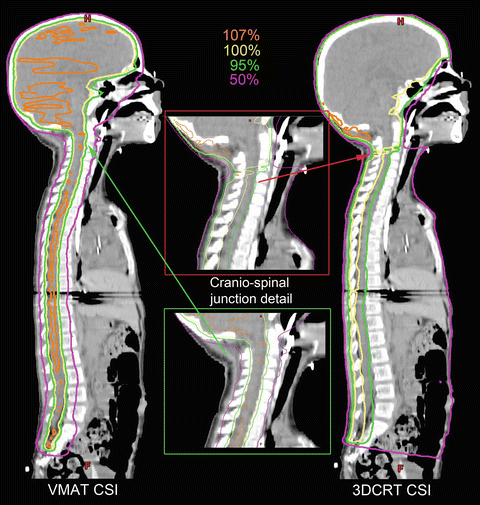
Fig. 22.4
Craniospinal irradiation dose distribution comparison between IMRT delivery (VMAT) and 3DCRT for a prescription of 23.4 Gy, with 6 MV photons, for a supine patient. IMRT plan is much more conformal, while the 3DCRT is more uniform. Furthermore, the junction between the cranial and spinal field is done dosimetrically with IMRT versus the classic dose feathering using match-moves in 3DCRT cases
Long-term follow-up of patients is important to prove that highly conformal plans which limit dose to critical structures translate to objective clinical reduction in late effects. Severe ototoxicity resulting in hearing loss has been a significant problem for children with medulloblastoma receiving radiotherapy, particularly when combined with cisplatinum-based chemotherapy. The boost dose to the posterior fossa using 3DCRT results in doses to the cochlea surpassing the prescribed dose of 54 Gy. Studies using audiometric tests as indicators for reduction in grade 3–4 hearing loss have been conducted in pediatric patients with brain tumors, including children with medulloblastoma treated with IMRT. A mean cumulative cochlear dose of less than 35 Gy is recommended after statistics indicated low incidence of ototoxicity with doses of 30 Gy or less and increased at greater than 40–45 Gy [61–63].
One of the most devastating effects of radiation therapy for medulloblastoma is impaired neurocognitive function, particularly for younger patients [64, 65]. Cumulative doses from standard 3DCRT to the entire posterior fossa result in substantial dose to the parietal, occipital, and temporal lobes, as well as the hypothalamic-pituitary axis. IMRT boost to the primary tumor site after standard CSI significantly reduces ototoxicities associated with 3DCRT techniques [66]. However, some have questioned whether this strategy may inadvertently increase the cumulative whole-brain doses due to the increased volume of exposure in the low dose range from the IMRT boost resulting in inferior neurocognitive outcomes. This hypothesis was tested by studying cognitive impairment in 25 patients with medulloblastoma treated with 3DCRT only versus IMRT used for the boost after standard CSI. There was no statistical difference between cohorts when assessing for long-term neurocognitive decline thus supporting the use of IMRT, which reduces normal tissues toxicities [21].
Studies have shown that the hippocampus, part of the temporal lobe, is involved with memory and is highly sensitive to radiation [67]. The degree of memory impairment associated with radiation therapy has been shown to be correlated to the mean hippocampal dose (relative to prescription dose). Investigators have systematically studied this using comprehensive standardized assessments of motor speed/dexterity, verbal memory, visual perception, vocabulary, and visual-spatial working memory and found a direct positive correlation between neurocognitive dysfunction and the amount of dose received by the subventricular zone, hippocampus, temporal lobes, and cerebrum [68]. Hippocampal-sparing IMRT techniques are being introduced into radiation therapy as a mechanism to minimize late neurocognitive effects. However, an unknown factor is whether this places patients at higher risk for relapse due to underdosing the adjacent ventricular system which may harbor tumor cells [69–72]. A specific detailed planning strategy is exemplified in the studies by Gondi et al. for hippocampal-sparing whole-brain radiotherapy using IMRT [73, 74]. Comparisons between 3DCRT and IMRT (standard and VMAT) have shown 12–23 % reduction in dose to the hippocampus with IMRT techniques with a corresponding reduction in the calculated risk for memory impairment. Prospective pediatric clinical trials would be important to validate this technique in the treatment of medulloblastoma.
Of note, in long-term follow-up of medulloblastoma patients, it is important to remember that transient imaging changes resembling leptomeningeal disease in the posterior fossa often occur on MRI surveillance specifically after IMRT boosts. To distinguish radiation-induced changes from recurrent disease, the timing of radiation therapy and location of boost are important features for the radiologist to be aware of, as these radiographic findings usually resolve within 6 months [75].
22.2.1.2 Glioma
Pediatric gliomas are histologically indistinguishable from their adult counterparts. However, clinical behavior and locations differ in the pediatric age group. Low-grade gliomas in young children are usually pilocytic astrocytoma tumors and found along the optic nerves, brainstem, and cerebellum. In older children, diffuse astrocytoma tumors more commonly arise in the cerebellum followed by cerebrum, deep midline structures, optic pathway, and brainstem. Low-grade gliomas are frequently cured with surgery. Radiotherapy is selectively administered for unresectable or progressive low-grade gliomas with doses of 50–54 Gy. Long-term cures are possible; therefore, highly conformal margins with IMRT are recommended to minimize late effects. A tight anatomically constrained expansion on the GTV (i.e., 5 mm margin to CTV) has been recommended as failure patterns are typically in the high-dose volume [76, 77].
Pediatric high-grade gliomas frequently occur in the brainstem where surgery is not feasible and prognosis is very poor. Radiation therapy is transiently effective but requires high doses of radiation therapy (59.4 Gy). The optimal margin for expansion on the GTV when using IMRT has not been established, but 1–1.5 cm margin to CTV has been used with the option for boost after 45 Gy if resection is not feasible.
22.2.1.3 Craniopharyngioma
Craniopharyngioma is a tumor arising in the sellar or suprasellar region from remnants of Rathke’s pouch with the highest incidence in the pediatric population occurring in those 5–14 years of age [78–80]. Radiation therapy is indicated for unresectable tumors, after partial resection, or recurrent disease. Doses of 54 Gy are prescribed using conformal margins for expansion on the solid and cystic component of the tumor of 0.5–1 cm margin to the CTV [81]. Determining target volumes for craniopharyngiomas is challenging as these tumors are prone to change during radiation therapy because of cystic components which are frequently associated with these tumors. Since IMRT is reliant on strict margins around GTV, any change in the tumor volume may under-/overdose the tumor or adjacent normal tissues. Close surveillance during treatment with MRI, CBCT, and repeat planning is recommended to account for observed tumor volume fluctuations, sometimes greater than 25 % [82].
22.2.1.4 Ependymoma
Ependymoma arises from the lining of the ventricular system with the highest incidence between 0 and 4 years of age, the fourth ventricle being the most common location. Postoperative radiation therapy is delivered after resection in selected patients with residual disease and/or high-grade tumors. Coverage to high doses (59.4 Gy) is frequently recommended for disease control as local recurrence is the predominant pattern of failure. Recommended margins for expansion include at most 1.5 cm margin for CTV from the operative bed and residual disease [83–85]. IMRT has been proven to increase the tumor control probability relative to 3DCRT and reduces dose to adjacent normal tissues. Given the possibility for daily image guidance and IMRT treatments, safe margin reductions to less than 1 cm have been recommended for future clinical trials to further minimization of normal tissue exposure [86].
Radiation therapy for ependymoma tumors, as well as for other tumors in close proximity to the hypothalamic-pituitary axis, increases the risk for associated growth hormone deficiency. Merchant et al. investigated the variation of growth hormone levels after radiotherapy in a cohort of children with ependymoma and demonstrated positive correlations between the time to develop growth hormone deficiency and cumulative dose to the hypothalamus. The authors determined that cumulative mean hypothalamic doses of 16 Gy are associated with a 50 % risk of developing growth hormone deficiency at 5 years [87]. Maximizing hypothalamic avoidance with IMRT delivery techniques is possible [88], inherently minimizing radiation therapy-associated growth deficiencies.
22.2.1.5 Germ Cell Tumors
Intracranial germ cell tumors (germinoma and non-germinomatous germ cell tumors) are rare, commonly occurring in the pineal and suprasellar region with a male preponderance. The role of IMRT for localized germinoma tumors is currently evolving toward limiting the extent of irradiation to the whole ventricular system, using doses in the range of 18–30.6 Gy followed by a conformal boost (23.4 Gy) to the primary site [89]. Compared to 3DCRT, whole ventricular system IMRT reduces the volume of normal brain irradiated in the higher isodose range (25–100 % of the prescription dose) by 0.7–16 %, without increasing the volumes irradiated in the lower dose component (5–10 %) [90]. VMAT delivery of IMRT for whole ventricle treatments can be utilized for more complex treatment geometries, delivering dose faster, with fewer monitor units, at the expense of increased low dose exposure of the normal brain [91]. Non-germinomatous germ cell tumors are less sensitive to radiotherapy. Traditional CSI treatments and prescription doses of 36 Gy with a boost to the primary site (up to 54 Gy) continue to play an important role in the disease control [92].
22.2.2 Sarcoma
Pediatric sarcomas comprise a diverse set of histologic subtypes occurring in children from infancy to adolescence and occur in all anatomic locations. Rhabdomyosarcoma (RMS) accounts for approximately 50 % of pediatric sarcomas. Other sarcomas are broadly categorized under the umbrella term: non-rhabdomyosarcoma soft tissue sarcomas (NRSTS). Synovial sarcoma and malignant peripheral nerve sheath tumor (MPNST) are among the most frequent histologic subtypes of NRSTS seen in children and young adults. Unlike RMS where a combination of surgery, chemotherapy, and radiation therapy remains the preferred treatment strategy, the surgery is the primary therapy for all NRSTS. Radiation therapy is selectively administered either postoperatively for high-grade tumors and positive margins or preoperatively for unresectable sarcomas or cases where radiotherapy is indicated based on size, grade, or location of the tumor [93]. For RMS, radiation doses range from 36–50.4 Gy depending on the extent of surgical resection, whereas NRSTS require higher doses. IMRT is the treatment of choice in pediatric sarcomas in most locations to achieve dose conformality while limiting normal tissues exposure.
Lin et al. reviewed the COG experience on dosimetric differences between IMRT and 3DCRT and whether there is an effect on local control for intermediate-risk pediatric patients with RMS. While dose coverage was improved with IMRT, there were no statistical differences between the rates of control between the two modalities [94]. Current pediatric oncology trials rely on multimodality imaging (MRI, PET-CT, or CT scans) obtained at the time of initial diagnosis to identify initial gross tumor volumes. Narrow margins are used for CTV expansion (1 cm) with a daily setup margin for uncertainty of 0.3–0.5 cm. Further refinements of the target volume can include field reduction to the post-induction chemotherapy volume for non-infiltrative tumors, using natural barriers, such as bone and skin, to define the limits of the CTV and shaping target volumes around critical structures that will surpass normal tissue dose constraints. Macdonald et al. reported on their experience with IMRT in the treatment of unresected pediatric head and neck RMS using limited margins and median doses of up to 50.4 Gy, with an option to reduce the treatment margins after an initial 36 Gy based on post-induction chemotherapy imaging studies. This strategy proved to be successful, providing excellent local control (100 % at 3 years follow-up) with significant normal tissue reductions particularly within the difficult-to-protect critical structures of the head and neck [95].
The optimal volumes and prescription dose levels have yet to be defined for NRSTS. A recent COG phase III NRSTS trial suggests that postoperative doses of 55.8 Gy for high-grade large tumors (>5 cm) yield very good local control for negative or microscopic positive surgical margins. In the preoperative setting, when combined with intensive chemotherapy, doses as low as 45 Gy have achieved excellent local control [96]. Krasin et al. prospectively studied the use of smaller margins when using IMRT and IGRT for pediatric NRSTS. Results indicated that using 2 cm anatomically constrained (bone, fascia) margins for CTV expansion on the GTV and an additional 0.4–1 cm for PTV expansion resulted in excellent local control at 3 years [97].
Sequential cone-down approaches have been shown to be beneficial for pediatric patients with sarcoma. IMRT planning for sequential boosts is a complicated process, since adequate target coverage with respect to normal tissue protection needs to be achieved for different plans, while the composite doses still need to be acceptable. This is a laborious process requiring a lengthy trial-and-error process or the use of complex optimization platforms [98]. DP and SIB are IMRT techniques which facilitate treatment of multiple target volumes to different prescription levels using the same number of fractions. This approach allows for better control of dose distribution by minimizing heterogeneity as compared to sequential cone-down techniques. The use of nonconventional dose per fraction has not been studied in the pediatric population. As fraction size has been demonstrated to be a critical radiation parameter in contributing to undesirable late effects, altered fractionation schemes would need to be tested in controlled clinical trial. Memorial Sloan Kettering Cancer Center reported a novel IMRT treatment approach for pediatric patients with sarcomas involving the thoracic region. While concomitantly delivering whole-lung or hemithorax lung irradiation for lung metastasis, DP IMRT was employed to treat the primary site. The recorded dose per fraction to the lungs was low (0.55–0.88 Gy), while the primary site received 1.8 Gy per fraction. A general decrease in the mean dose to the esophagus (15 %), heart (31 %), spinal cord (15 %), and liver (19 %) was observed using the proposed DP technique compared to standard techniques, with no local failures [35].
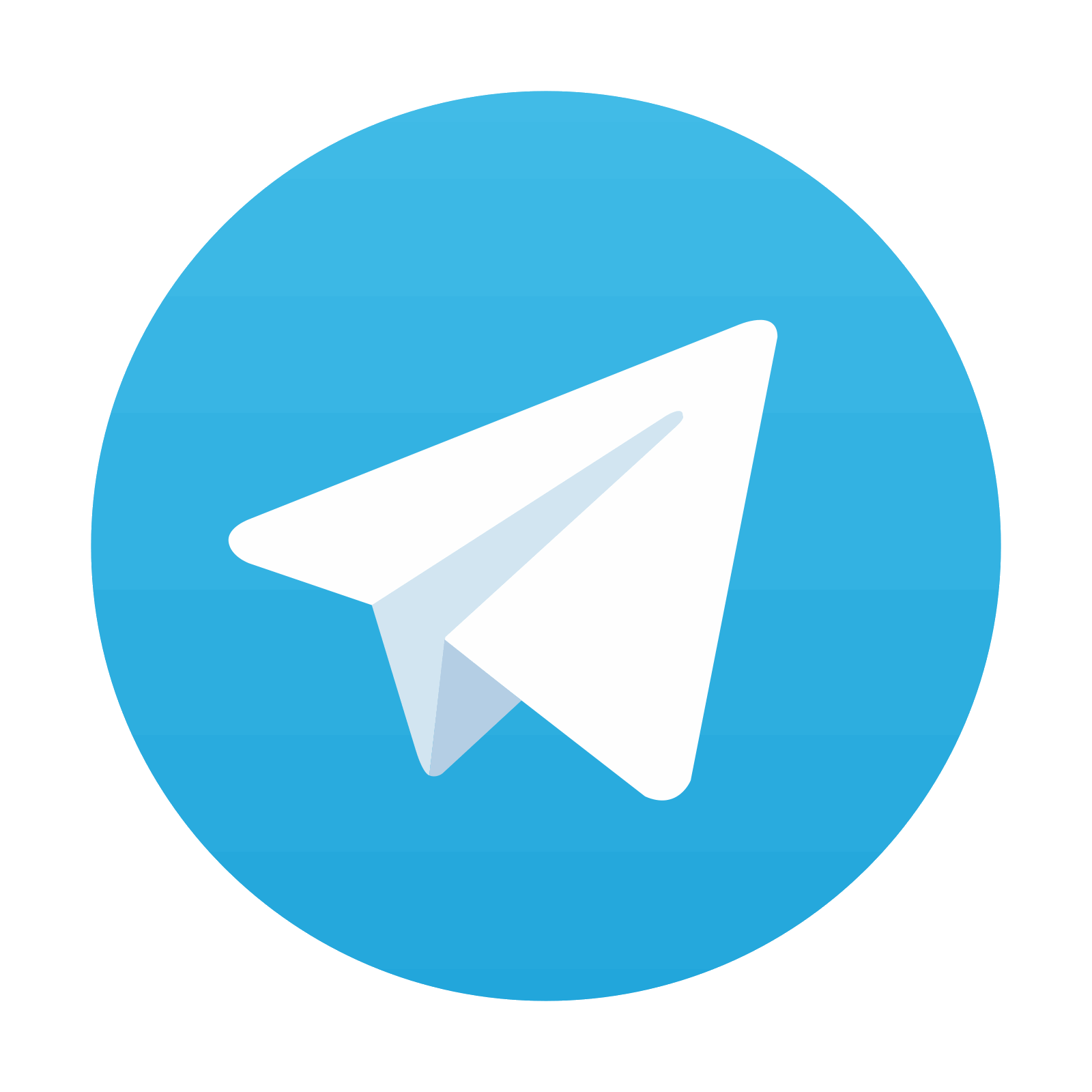
Stay updated, free articles. Join our Telegram channel
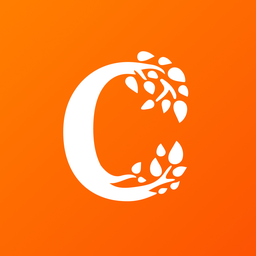
Full access? Get Clinical Tree
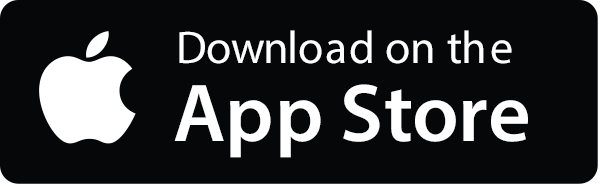
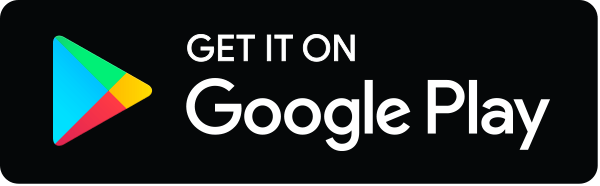