(1)
Department of Nuclear Medicine, Kuwait University, Safat, Kuwait
7.1 Thyroid Gland
7.1.3 Major Thyroid Disorders
7.2.2 Hyperparathyroidism
7.2.3 Parathyroid Adenoma
7.2.4 Parathyroid Hyperplasia
7.2.5 Parathyroid Carcinoma
7.3 Adrenal Gland
7.3.2 Adrenal Cortex
7.3.3 Adrenal Medulla
7.3.4 Incidental Adrenal Mass
Abstract
The thyroid gland develops from the foramen cecum of the tongue, to which it is connected by the thyroglossal duct. It descends during fetal life to reach the anterior neck by about the seventh week. Absent or aberrant descent results in ectopic locations, including the sublingual region and superior mediastinum.
7.1 Thyroid Gland
7.1.1 Anatomical and Physiological Considerations
7.1.1.1 Thyroid Anatomy
The thyroid gland develops from the foramen cecum of the tongue, to which it is connected by the thyroglossal duct. It descends during fetal life to reach the anterior neck by about the seventh week. Absent or aberrant descent results in ectopic locations, including the sublingual region and superior mediastinum.
The normal adult thyroid gland weighs 14–18 g. It is generally smaller in women than in men and is barely palpable [1, 2]. The thyroid is located in the mid to lower anterior neck, with the isthmus in front of the trachea, usually just below the cricoid cartilage, and the lobes on the sides of the trachea (Fig. 7.1). In older individuals with shorter necks, the thyroid may lie at or just above the suprasternal notch, and it may often be partly substernal. The thyroid gland moves cephalad during swallowing, a characteristic that aids in palpation and in distinction of the thyroid from non-thyroid neck masses.
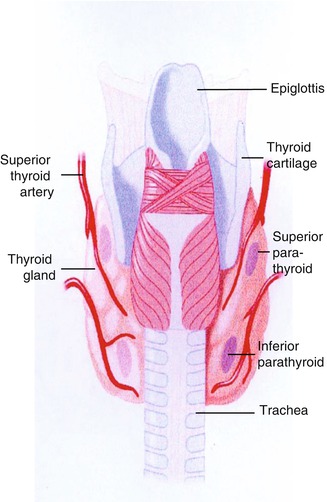
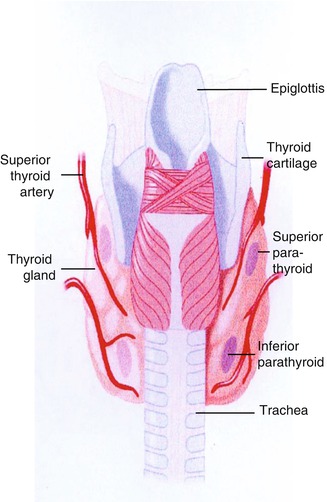
Fig. 7.1
Diagram showing typical locations of the thyroid and parathyroid glands
7.1.1.2 Hormone Synthesis and Secretion
The thyroid follicle consists of a colloid center, which acts as a storage site for thyroid hormone, surrounded by epithelial cells (Fig. 7.2). The thyroid epithelial cell has a transport mechanism, also referred to as trapping, which enables thyroid concentration of iodide [3, 4]. A plasma membrane protein, the sodium/iodide symporter (NIS), is responsible for iodide transport. Symporter activity is influenced primarily by the pituitary thyroid-stimulating hormone (TSH), which increases the transport of iodide. The trapped iodide subsequently undergoes organification and incorporation into thyroid hormones.
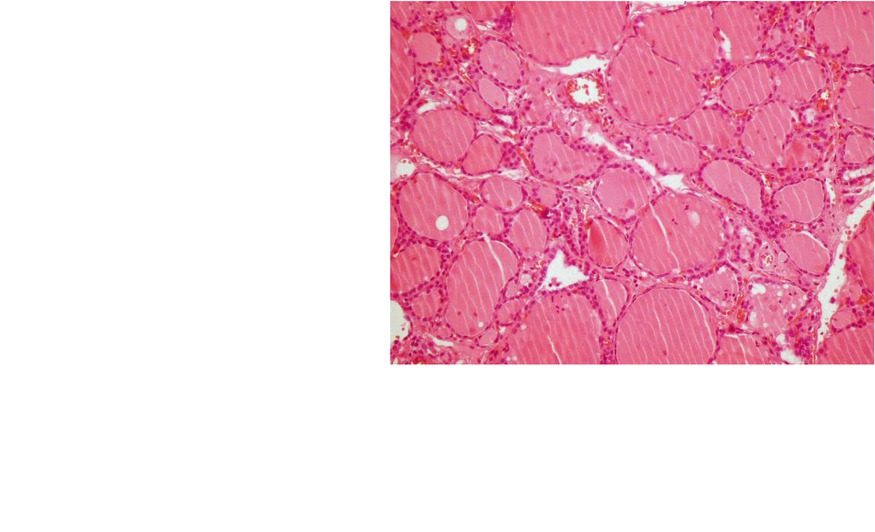
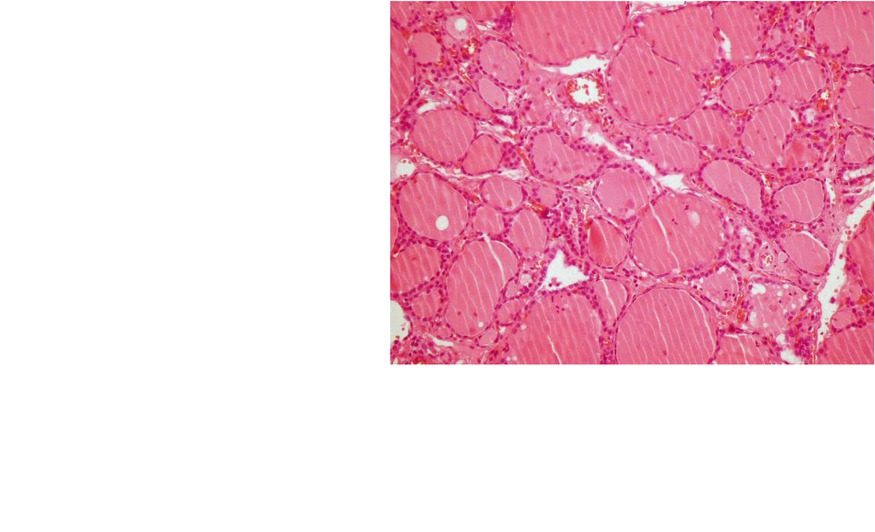
Fig. 7.2
Thyroid follicle. Low-power field images of thyroid gland tissue showing the follicles lined by follicular cells and filled with colloid
Other anions, including pertechnetate, thiocyanate, and perchlorate, also are accumulated by the thyroid gland. The uptake of pertechnetate is the basis for 99mTc-pertechnetate scintigraphy. Thiocyanate, derived from certain foods, decreases thyroid accumulation of iodine and may exacerbate iodine deficiency. Perchlorate has diagnostic and therapeutic applications.
Synthesis of hormone takes place in the thyroglobulin, a glycoprotein, which is produced in the thyroid cell and extruded into the colloid. Iodine combines with tyrosine in thyroglobulin to form monoiodotyrosine (MIT) and diiodotyrosine (DIT). Subsequently, the iodotyrosines are coupled, with the formation of thyroxine (T4) and triiodothyronine (T3). The coupling reaction also is mediated by peroxidase.
In response to TSH, a small amount of colloid is engulfed by the epithelial cell and proteolyzed, with release of T3 and T4, which diffuse into the circulation. Thyroglobulin not undergoing proteolysis also enters the circulation in small quantities. The serum thyroglobulin has been used as a tumor marker in differentiated thyroid cancer. Thyroglobulin decreases and eventually becomes undetectable following thyroidectomy and 131I ablation, and its subsequent rise indicates a recurrence. TSH stimulation, by promoting colloid endocytosis, increases the amount of thyroglobulin released. Consequently, the serum thyroglobulin is a more reliable tumor marker at high TSH levels [5, 6].
Most of the circulating thyroid hormones are bound to plasma proteins, the free fraction comprising about 0.05 % of T4 and 0.2 % of T3. Only the free hormone has metabolic effects, and it is a more accurate measure of thyroid function than the total hormone, which varies with plasma proteins levels.
T3 is considered the active hormone. About 20–30 % of the circulating T3 is secreted by the thyroid gland, and the remainder is produced by monodeiodination of T4 in extrathyroid tissues, notably the liver, kidney, brain, and pituitary [6]. Decrease in the peripheral conversion of T4 to T3 is a basis for the use of some antithyroid drugs.
Most antithyroid drugs generally block one or more steps in the synthesis and metabolism of thyroid hormone (Table 7.1). The thiourea derivatives (thionamides), including propylthiouracil (PTU) and methimazole, are the most common antithyroid agents in use [7, 8]. Methimazole is generally the preferred drug since PTU may be associated with serious hepatotoxicity [8]. Other drugs used for their antithyroid actions include glucocorticoids, iodides, lithium, and potassium perchlorate [6].
Table 7.1
Actions and uses of antithyroid drugs
Drug | Mechanism | Uses |
---|---|---|
Propylthiouracil (PTU) | Blocks iodine organification | Controls hyperthyroidism in Graves’ disease and toxic nodular goiter |
Decreases the monodeiodination of T4 to T3 | ||
Decreases in serum levels of thyrotropin receptor autoantibodies | ||
Methimazole | Blocks iodine organification | Controls hyperthyroidism in Graves’ disease and toxic nodular goiter |
Decreases in serum levels of thyrotropin receptor autoantibodies | ||
Glucocorticoids | Rapid inhibitory effect on peripheral conversion of T4 to T3 | Thyroid storm, Graves’ ophthalmopathy, and protracted subacute thyroiditis |
Iodides | Decreases synthesis of thyroid hormones | Thyroid storm |
Lithium | Blocks release of thyroid hormone | Adjunct in treatment of severe hyperthyroidism |
Potassium perchlorate | Decreases thyroid iodine uptake and discharges unbound iodine | Amiodarone-induced thyroid dysfunction and after accidental exposure to radioactive iodine |
7.1.1.3 Thyroid-Stimulating Hormone (TSH)
Thyrotropin-releasing hormone (TRH), a tripeptide originating from the hypothalamic median eminence, stimulates the secretion and synthesis of thyroid-stimulating hormone (TSH, thyrotropin), a glycoprotein, by the anterior pituitary. It increases the transport of iodide, synthesis of hormone, and release of T3, T4, and thyroglobulin. The production and release of TSH are influenced by the concentration of T3 within the pituitary. When the T3 concentration falls below a set point, TSH secretion increases, and synthesis and release of thyroid hormones are accelerated. Conversely, when the T3 level rises above the set point, TSH release is inhibited. In addition to its pituitary effect, T3 inhibits hypothalamic TRH release. Other mechanisms reported more recently include the inhibitory actions of the released TSH on TRH secretion and on TSH receptors in the pituitary itself.
In addition to regulation of thyroid function, TSH promotes thyroid growth. If thyroid hormone synthesis is chronically impaired, as in iodine deficiency and autoimmune thyroid disease, chronic TSH stimulation eventually may lead to the development of a goiter.
The serum TSH is a sensitive and specific marker of thyroid function. Normal serum TSH is about 0.45–4.5 μunits/ml, and levels up to 20 μunits/ml are considered normal in newborns because of the contribution of maternal TSH. Serum TSH is increased in primary hypothyroidism and decreased in hyperthyroidism of all etiologies except for the uncommon entity of thyrotropin-induced hyperthyroidism.
The TSH secretion is suppressed by exogenous thyroid hormone to avoid stimulation of tumor growth in patients with differentiated thyroid cancer and to decrease thyroid size or arrest thyroid growth in the early stages of goiter development.
Stimulation with TSH increases thyroid function and thyroid uptake of radioiodine. This principle is used in differentiated thyroid cancer for the detection and treatment of thyroid remnants and thyroid cancer metastases with radioiodine [5, 9]. Thyroid-stimulating hormone levels are allowed to rise to 30–50 μunits/ml or higher after withholding thyroid hormone supplements or after administering recombinant human TSH. The latter is becoming popular since it shortens the preparation time and avoids a period of hypothyroidism after stopping thyroid hormone replacement therapy [9–11]. In thyroid cancer, recombinant TSH is used in diagnosis and monitoring of serum thyroglobulin and for radioiodine ablation of thyroid remnants after thyroidectomy. FDG PET is optimal at high TSH levels, and it may be combined with radioiodine imaging and thyroglobulin measurement in selected patients [12–14].
7.1.1.4 Thyroid Function and Iodine Intake
7.1.1.4.1 Iodine Deficiency
The daily requirement for iodine is about 150 μg, increasing to roughly 200–250 μg during pregnancy. Reduced synthesis of thyroid hormone is compensated, at least in part, by increased TSH secretion, resulting eventually in goiter formation.
7.1.1.4.2 Iodine Excess
When intrathyroid iodine concentrations are significantly increased, the rate of thyroid hormone synthesis is decreased, with a reduction in iodothyronine synthesis and decrease in the DIT/MIT ratio. This response is referred to as the Wolff-Chaikoff effect.
Continued exposure to large amounts of iodine would eventually lead to hypothyroidism, with compensatory increase in TSH and development of goiter. While this does occur occasionally, adaptation or escape from the effects of chronic iodide excess is more likely. The inhibitory effect of iodides on thyroid function is utilized clinically for prompt control of severe hyperthyroidism and thyroid storm. In Graves’ disease, large doses of iodide decrease not only hormone synthesis but also hormone release [15]. Since escape from the inhibitory effect is likely, iodide therapy is only a short-term measure for lowering thyroid hormone levels rapidly.
Iodine excess may lead to hyperthyroidism [16, 17]. Iodine-induced hyperthyroidism, referred to as Jod-Basedow, characteristically occurs in persons with hyperplastic thyroid glands. Hyperthyroidism occurring after iodine supplementation in endemic goiter areas is a classical example. Iodine-containing medical products, including amiodarone, radiographic dyes, and kelp, also have the potential to cause Jod-Basedow [18–20]. Amiodarone, a cardiac antiarrhythmic drug, is probably the most common source of iodine today. Each 200 mg tablet yields about 7 mg free iodine, while the daily requirement is only 0.15 mg. Amiodarone-related hyperthyroidism may be related to another mechanism. The drug may cause thyroiditis.
7.1.2 Thyroid Radiopharmaceuticals
7.1.2.1 Technetium-99m-Pertechnetate
Technetium-99m-pertechnetate is widely used for imaging the thyroid gland. It is trapped by the thyroid, but unlike iodine, it does not undergo organification and remains in the gland for a relatively short duration. Therefore, imaging is done about 20–30 min after intravenous administration of the radiotracer. Approximately 5–10 mCi (185–370 MBq) is used. The thyroid-to-background activity ratio is not as high as that with radioiodine, so that 99mTc-pertechnetate is unsuitable for imaging to search for metastatic thyroid carcinoma, which usually functions poorly compared with normal thyroid tissue. Imaging of ectopic mediastinal thyroid tissue also may be suboptimal due to high blood and soft tissue background activity.
7.1.2.2 Iodine-123
Iodine-123 has ideal characteristics for imaging the thyroid gland, with a short physical half-life of 13 h, absence of beta emissions, and high uptake in thyroid tissue relative to background [20]. However, it is less readily available and more expensive than 99mTc-pertechnetate. 123I undergoes organic binding in the thyroid gland, and imaging is usually done 4–24 h after oral administration of 200–400 μCi (7.4–14.8 MBq) of radiotracer. Because of its superior biodistribution characteristics, 123I is preferred over 99mTc-pertechnetate for imaging of poorly functioning and ectopic thyroid glands. 123I also may be used for whole-body imaging in differentiated thyroid cancer. Approximately 2–4 mCi (74–148 MBq) of the radiotracer is used for this purpose.
7.1.2.3 Iodine-131
Iodine-131 may be used for the measurement of thyroid uptake, which requires only very small amounts of radiotracer. It is no longer used for routine imaging of the thyroid gland but continues to be valuable for the detection of metastases and recurrences in differentiated thyroid cancer [5, 21, 22]. Following appropriate patient preparation to increase TSH levels, 2–4 mCi (74–148 MBq) of 131I is administered orally, and imaging is performed 48–96 h later. Radioiodine imaging has diagnostic as well as prognostic value. Iodine-avid tumors tend to be well-differentiated histological features and a favorable prognosis, whereas tumors that do not accumulate iodine are likely to be less differentiated and more aggressive [5, 23, 24].
Iodine-131 delivers a high radiation absorbed dose to the thyroid, with relative sparing of non-thyroid tissues. It is therefore ideal for the treatment of thyroid disease and used extensively in the management of Graves’ disease, toxic nodular goiter, and differentiated thyroid cancer.
7.1.2.4 Fluorine-18Fluorodeoxyglucose (18F-FDG)
Positron emission tomography (PET) with 18F-FDG is used in evaluating a variety of neoplasms including differentiated thyroid cancer. In differentiated thyroid cancer, FDG may be used to identify metastases not visualized at radioiodine imaging and to assess prognosis. Lesions that accumulate FDG tend to follow a more aggressive course than lesions that are not FDG-avid [12]. Whole-body FDG PET, therefore, is useful in evaluating high-risk thyroid cancer. Patient preparation is similar to that for radioiodine scintigraphy, since the uptake and diagnostic sensitivity of FDG are increased by TSH stimulation [13, 14]. Focal uptake of FDG within the thyroid gland, an occasional finding at evaluation of non-thyroid cancers, may be related to a benign or malignant pathology [24, 25].
7.1.3 Major Thyroid Disorders
7.1.3.1 Nodular Thyroid Disease
7.1.3.1.1 Pathophysiology
Thyroid nodules are common. In the United States, where iodine deficiency has been corrected by iodine supplements, thyroid nodules are clinically detectable in 4–7 % of the population [26, 27]. The prevalence is greater in countries affected by iodine deficiency. The incidence of thyroid nodules in apparently normal thyroid glands is greater than 50 % in autopsy series.
Most thyroid nodules are benign, particularly in multinodular goiter. Malignancy in clinical thyroid nodules is reported to occur in 5–20 % of nodules. Mortality due to thyroid cancer is generally very low.
Types of Thyroid Nodule
Thyroid nodules represent a wide spectrum of thyroid diseases. In a normal-sized gland or a diffuse goiter, thyroid nodules may be solitary or multiple. In multinodular goiters, a nodule may become clinically dominant in terms of growth, dimensions, and functional characteristics. A clinicopathological classification of thyroid nodules subdivides nodules into nonneoplastic nodules, true neoplastic nodules (benign or malignant), and micronodules.
Nonneoplastic Nodules (Pseudo-nodules)
These nodules may be seen in patients with thyroid hyperplasia and inflammatory or autoimmune thyroid diseases:
(a)
Glandular hyperplasia arising spontaneously or following previous partial thyroidectomy
(b)
Rare forms of thyroid hemiagenesis which may present as hyperplasia of the existing thyroid tissue, mimicking a thyroid nodule
(c)
Hashimoto’s thyroiditis associated nodules, indicative of lymphocyte infiltration
(d)
Nodules found during the initial phase of subacute thyroiditis, resulting from the inflammatory process
Neoplastic Nodules
Based on scintigraphy, thyroid nodules are classified into functioning (hot) nodules which are able to concentrate radioactive iodine or technetium-99 m-pertechnetate and nonfunctioning (cold) which show radiotracer uptake less than that in normal thyroid tissue. Hot nodules represent from 3 to 20 % of thyroid nodules, according to the geographical origin of the patients as their incidence is higher in countries where iodine deficiency is still present. They are three to four times more frequent in females and tend to occur in those older than 40 years. In the great majority of cases, hot nodules are benign. Cold nodules account for more than 80 % of all thyroid nodules. Three types of nodule are distinguished by ultrasonography: cystic, solid, and mixed (containing solid and cystic components). Cystic nodules (10–20 % of all nodules) are almost always benign. Thyroid cancer is found in approximately 10 % of cold nodules (solitary and multiple) that are solid or mixed at ultrasonography. More than 75 % of malignant nodules are differentiated thyroid cancer of the follicular epithelium (papillary and follicular) with excellent prognosis. The other types of cancer are rare and include anaplastic or undifferentiated carcinoma (2–14 % of all thyroid carcinomas) and medullary thyroid carcinoma representing 5–10 % of thyroid carcinomas and originating from the calcitonin-producing parafollicular C cells of the thyroid [28, 29]. Lymphoma and metastases to thyroid are other uncommon malignancies.
Micronodules
Micronodules describe nodules of 1 cm or less in diameter. These nodules are discovered increasingly by the commonly used ultrasonography. In the absence of other suspicious clinical criteria, they only require to be followed by repeated thyroid ultrasonography.
7.1.3.1.2 Scintigraphy
When thyroid nodule is discovered, the main problem is distinguishing between a benign and a malignant lesion. This problem has largely been solved by fine-needle biopsy which makes that distinction when performed by experienced cytologists. Accordingly, the role of thyroid scintigraphy is reserved to the assessment of the functional activity of the nodules and when functional autonomy is suspected (Figs. 7.3, 7.4, and 7.5). Thyroid scintigraphy is performed then when serum TSH is suppressed or low in a patient with a single nodule and in all patients with multinodular goiter, regardless of the TSH result. Iodine-123 and 99mTc-pertechnetate are routinely used for imaging thyroid nodular disease. 18F-FDG PET has low specificity (63 %) in the diagnosis of thyroid nodules but can help to select patients who need surgery when cytology is inconclusive in view of its high (100 %) negative predictive value for thyroid cancer [30].
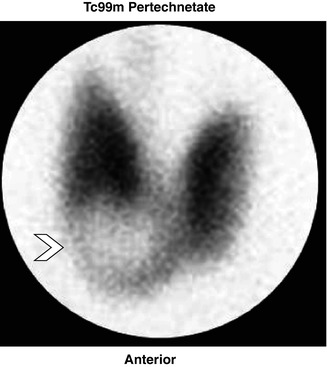
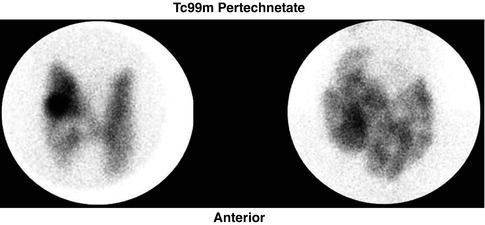
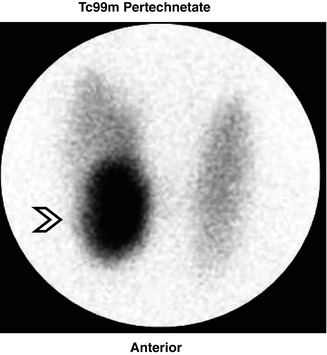
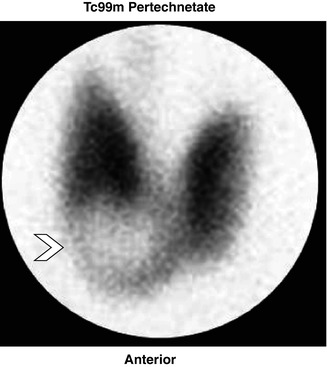
Fig. 7.3
Solitary nodule. 88mTc-pertechnetate thyroid scan anterior pinhole image showing a large solitary cold nodule (arrow)
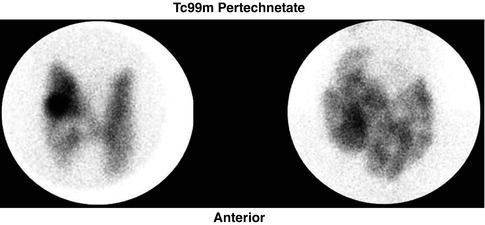
Fig. 7.4
Two examples of multinodular goiter as it appears on scintigraphy. (a) represents a mixture of cold and hot nodules, while (b) shows multiple cold nodules
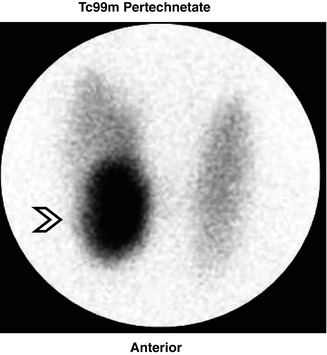
Fig. 7.5
A study showing a toxic nodule in the right lower lobe (arrow) with suppression of the remainder of the gland
Whole-body 131I and FDG PET have a role in the investigations and follow-up of thyroid cancer (see Chaps. 10 and 14). On FDG PET scans, a normal thyroid gland demonstrates absent or low-grade FDG uptake. FDG PET may incidentally identify thyroid uptake. In general, a diffuse uptake by the thyroid gland is considered to be benign and very likely secondary to thyroiditis and/or hypothyroidism, while a focal uptake of the thyroid on FDG PET is defined as an incidentaloma, which is more clinically significant due to its high risk of malignancy ranging from 25 to 50 % [31–33].
7.1.3.2 Destructive Thyroiditis
Destructive thyroiditis, also referred to as subacute thyroiditis, is characterized by cell membrane breakdown and consequently release of excessive amounts of thyroid hormone into the circulation. The usual causes are autoimmune thyroid disease, viral infection, and amiodarone treatment. Less commonly, thyroiditis may be related to treatment with certain drugs such as interferon alpha, interleukin-2, lymphokine-activated killer (LAK) cells, and lithium. These therapeutic agents probably exacerbate existing autoimmune thyroid disease [34–36]. Bacterial thyroiditis is currently rarely seen.
Thyroiditis typically painful usually resolves spontaneously. Hyperthyroidism in the active phase is followed by transient hypothyroidism before restoration of the euthyroid state, usually in 6–12 months. Treatment usually consists of β-adrenergic blockers in the hyperthyroid phase and analgesics for pain. Protracted thyroiditis may however require glucocorticoids.
7.1.3.2.1 Postpartum Thyroiditis
Postpartum thyroiditis, also known as painless or subacute lymphocytic thyroiditis, is the principal thyroid disorder in postpartum women. It may be considered an accelerated form of autoimmune thyroid disease, attributed to suppression of immune-related disorders during pregnancy with a rebound after childbirth [37–39]. For the same reason, Graves’ disease also may occur in the postpartum period, though less frequently, and a strong association with insulin-dependent diabetes mellitus, an autoimmune condition, has been noted.
Postpartum thyroiditis, like other forms of destructive thyroiditis, is self-limited but tends to reoccur in subsequent pregnancies. Permanent hypothyroidism occurs in 20–25 % of patients over a period of 5 years. Elevated thyroid peroxidase (anti-microsomal) antibodies during pregnancy are associated with a sharp increase in postpartum thyroiditis.
7.1.3.2.2 Viral Thyroiditis
Viral subacute thyroiditis, also known as de Quervain’s thyroiditis, usually occurs after an upper respiratory tract infection. The disorder tends to be seasonal and may occur in clusters, occasionally causing mini epidemics [40]. It usually presents as a painful and tender goiter, associated with general malaise and possibly fever. Inflammation frequently begins in one lobe of the thyroid and gradually spreads to involve the entire gland. Permanent hypothyroidism is uncommon.
7.1.3.2.3 Amiodarone-Induced Thyroiditis
Amiodarone is an iodine-rich benzofuran derivative used to treat and prevent cardiac arrhythmias. It may precipitate a number of thyroid conditions including thyroiditis, which appears to be related to a cytotoxic effect [18, 19]. Thyroid hormone synthesis may increase or decrease. Other actions of amiodarone include blocking peripheral conversion of T4 to T3, binding of T3 to its receptors, and thyroid release of T3 and T4. These effects may permit the use of amiodarone in very selected cases of hyperthyroidism [41].
Since amiodarone and its metabolite desethylamiodarone have long half-lives of up to 100 days, the thyroid-related effects can be protracted and occasionally may begin after stopping the drug. Amiodarone-induced thyroiditis generally requires treatment with a glucocorticoid. Permanent hypothyroidism is uncommon.
7.1.3.3 Autoimmune Thyroid Disease
7.1.3.3.1 Etiological Factors
Autoimmune thyroid disease comprises two major entities, Hashimoto’s disease (chronic autoimmune thyroiditis) and Graves’ disease. Variants of Hashimoto’s disease include subacute thyroiditis, which occurs typically in the postpartum period, and atrophic thyroiditis. There is a genetic predisposition to the disease, with contribution from environmental factors [42–45] (Table 7.2).
Table 7.2
Etiological factors for autoimmune thyroid disease
Genetic predisposition |
Iodine excess |
Cigarette smoking |
Female gender |
Psychological stress |
Infection |
7.1.3.3.2 Pathophysiology
Hashimoto’s Disease
Elevation of thyroid peroxidase antibodies is characteristic of Hashimoto’s disease. Antithyroglobulin antibodies may also be elevated. Hormone synthesis is impaired with immune thyroid disease compensatory increase in TSH secretion, which stimulates thyroid function and growth. Eventually, many patients become hypothyroid. Both overt and subclinical hypothyroidism related to autoimmune disease are widely prevalent in iodine-sufficient regions [46]. Exacerbation of Hashimoto’s disease, frequently occurring in the postpartum period, is a cause of subacute thyroiditis.
Graves’ Disease
Graves’ disease is associated with high levels of thyrotropin receptor autoantibodies (TRAB) that stimulate thyroid growth and thyroid hormone synthesis and release [43, 47]. Most organ systems are affected by Graves’ disease, the cardiovascular manifestations being the most apparent. Increased heart rate and contractility increase the cardiac output. These effects are related to a direct inotropic effect of T3, decreased systemic vascular resistance, increased preload related to a higher blood volume, and heightened sensitivity to sympathetic stimulation. Blood volume is increased by activation of the renin-angiotensin-aldosterone system caused by the reduction in systemic vascular resistance and by increased erythropoietin activity. Overt cardiac failure may result from severe and prolonged hyperthyroidism but is rarely seen today. Atrial fibrillation is not an uncommon complication, occurring in up to 15 % of patients with hyperthyroidism [48, 49].
Scintigraphy
Thyroiditis
Poor radioiodine/99mTc-pertechnetate uptake in the thyroid gland is the hallmark of subacute thyroiditis of any etiology (Fig. 7.6). Decreased tracer uptake is related to TSH suppression by excessive thyroid hormone released from damaged follicles and to decreased hormone synthesis in the damaged gland. The thyroid uptake and scan normalize with resolution of thyroiditis.
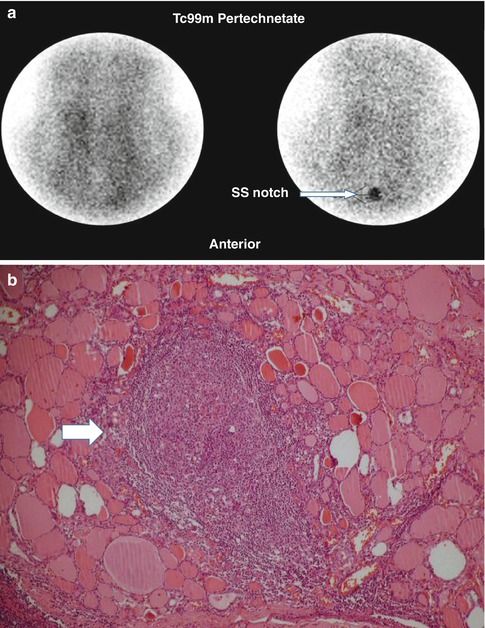
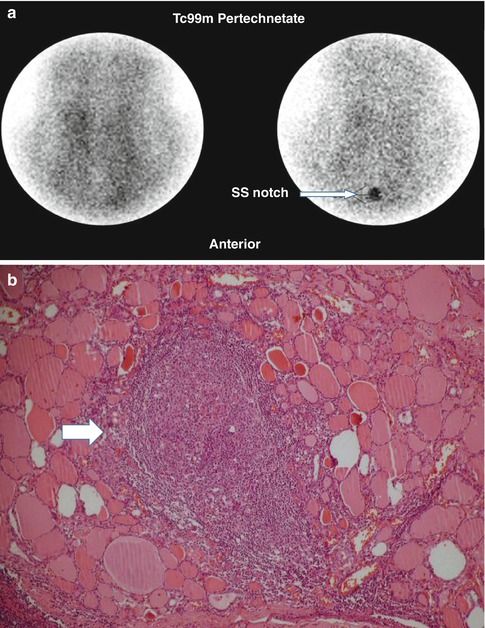
Fig. 7.6
Thyroiditis (a, b). (a) 99mTc-pertechnetate thyroid scan in an 8-year-old girl with a recent history of upper respiratory infection. The scan shows poor uptake by the thyroid gland with poor delineation of its outlines. The thyroid uptake with 0 %. (b) Microscopic illustration of thyroiditis with a focus of inflammatory cells (arrow) surrounded by thyroid follicles (Courtesy of Professor Magda El-Monayeri with thanks)
Scintigraphy is frequently used in some hyperthyroid patients to differentiate autoimmune thyroiditis, with low uptake, from Graves’ disease, with high uptake [50].
A thyroid uptake/scan may be worthwhile in amiodarone-related hyperthyroidism, which may be due to Jod-Basedow or thyroiditis. A low thyroid uptake is frequently found in patients treated with amiodarone and is nondiagnostic, while a normal or high uptake suggests that Jod-Basedow is likely. The thyroid uptake measurement also helps determine the feasibility of 131I treatment in refractory cases.
Scintigraphy is nonspecific in Hashimoto’s disease. The thyroid gland is usually symmetrically enlarged with uniform tracer distribution, and the 24-h uptake is normal. In subacute thyroiditis resulting from exacerbation of Hashimoto’s disease, tracer uptake is typically absent or very low.
Graves’ Disease
Graves’ disease typically shows uniformly increased tracer uptake in a diffusely enlarged thyroid gland, frequently with visualization of a pyramidal lobe (Fig. 7.7) and decreased background activity of various degrees based on the severity of the condition. However, atypical appearances, particularly in Graves’ disease superimposed on nodular goiter, are occasionally encountered. If needed, TRAB measurement may assist in confirming the diagnosis. The 24-h uptake is elevated and, typically, much higher than in toxic nodular goiter [51, 52].
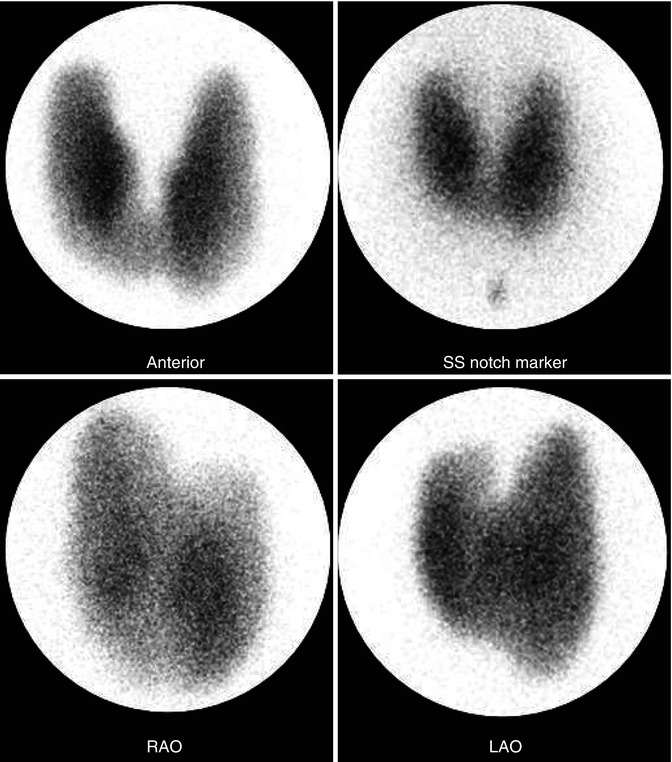
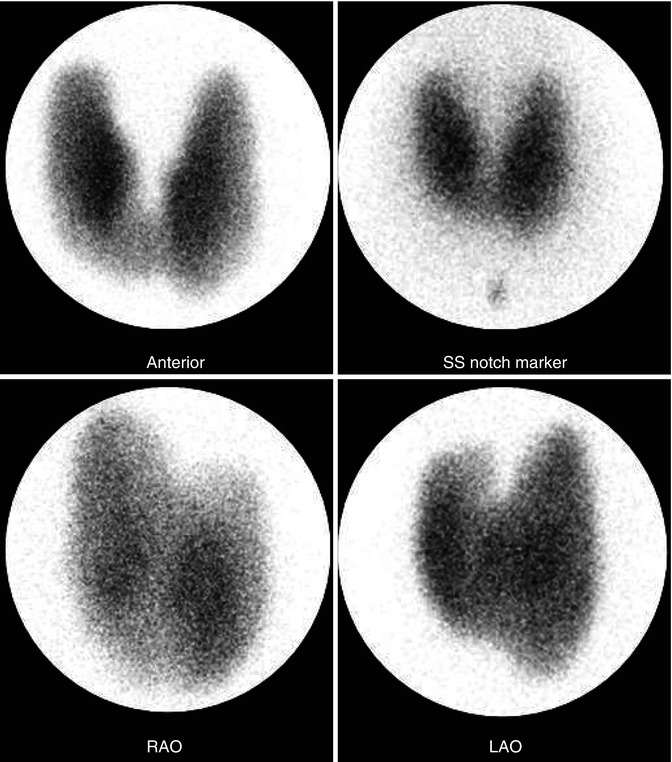
Fig. 7.7
Graves’ disease new. 99mTc-thyroid scan illustrating the pattern of Graves’ disease. There is uniform radionuclide distribution with low background activity
7.1.4 Thyroid Dysfunction During Pregnancy
Hyperthyroidism in pregnancy is generally caused by gestational transient thyrotoxicosis (GTT) or Graves’ disease. Management of Graves’ disease remains a challenge, with thionamide treatment as the best option. Patients should be monitored closely because undertreatment, with persistently high maternal TRAB, increases the risk of fetal hyperthyroidism, while overtreatment may cause fetal hypothyroidism. Gestational hypothyroidism is usually related to autoimmune thyroid disease and less frequently to iodine deficiency. The latter may be associated with fetal hypothyroidism as well.
7.2 Parathyroid Gland
7.2.1 Anatomical and Physiological Considerations
Normal parathyroid glands are derived from the pharyngeal pouches, the upper glands from the endoderm of the fourth pouch and the lower glands from the third pouch. The parathyroid glands are typically located on the thyroid gland (Fig. 7.1). Occasionally one or more glands may be embedded in the thyroid. The normal position of the superior parathyroids is at the cricothyroid junction, above the anatomical demarcation of the inferior thyroid artery and the recurrent laryngeal nerve [53]. The inferior parathyroids are more widely distributed mostly anterolateral or posterolateral to the lower thyroid gland [54]. The accessory glands that can be variously located in humans, from the cricoid cartilage down into the mediastinum [55, 56], are derived from the numerous dorsal and ventral wings of the pouches. Normally, human beings have four glands, but more or fewer than this number are found in some individuals [57]. Among healthy adults, 80–97 % have four parathyroids; approximately 5 % have fewer than four glands, and 3–13 % have supernumerary glands [54].
The normal glands usually measure 4–6 mm in length, 2–4 mm in width, and 0.5–2 mm in thickness. The glands are usually ovoid or bean shaped but may be elongated, flattened into a leaflike structure, or multilobulated [58]. The weight of the glands is usually 30 mg each, with the largest normal gland not exceeding 70 mg. The total weight of the glands is less than 210 mg, and the total parenchymal cell weight is less than 145 mg [69]. In normal glands, parenchymal cells are predominantly chief cells which contain cytoplasmic fat droplets. Oxyphilic and transitional oxyphilic cells are sparsely present in children and young adults and increase to 4–5 % of the parenchymal cells in old age. These cells tend to form nodules if they increase in number and have a very small amount of fat or no fat at all in their cytoplasm. Ultrastructurally, oxyphil cells are characterized by the presence of closely packed mitochondria, while chief cells contain moderate to high mitochondrial content. Water-clear cells are vacuolated with distended organelles. Each of the three cell types may contain varying amounts of lipid droplets and residual bodies. Table 7.3 summarizes the types of parathyroid cells and their function.
Table 7.3
Cells of the parathyroid glands and their functions
Cell type | Major ultrastructural feature | Function |
---|---|---|
Chief cell | Slightly eosinophilic cytoplasm,few mitochondria | The active endocrine cell, producingthe parathyroid hormone |
Oxyphil cell | Rich eosinophilic cytoplasm,tightly packed mitochondria | May be able to produce parathyroidhormone |
Transitional oxyphil cell | Less eosinophilic cytoplasm | Variant of oxyphil cell |
Clear cell | Foamy and water-clear cytoplasm | Unknown, fundamentally inactive |
Parathyroid hormone has four principle actions: (a) to increase calcium absorption from the gastrointestinal tract; (b) to stimulate osteoclastic activity, resulting in resorption of calcium and phosphate from bone; (c) to inhibit phosphate reabsorption by the proximal renal tubules; and (d) to enhance renal tubular calcium reabsorption. Parathyroid hormone secretion is controlled mainly by the extracellular calcium concentration. The parathyroid cell surface is thought to be equipped with a cation-sensitive receptor mechanism through which ambient calcium regulates the cytosolic calcium (Ca2+i) concentration and parathyroid hormone secretion. Activation of this receptor causes also activation of protein kinase C [58]. 1,25-Dihydroxycholecalciferol reduces the secretion of parathyroid hormone independent of any changes in calcium concentration. Parathyroid hormone is metabolized in Kupffer’s cells of the liver.
In patients with hyperparathyroidism, pathological parathyroid cells show defective sensing of ambient calcium. The cellular basis of this abnormality is unknown, although increased protein kinase C activity within abnormal parathyroid cells may be the mechanism. Pathological parathyroid glands also have an increased parenchymal cell content, although the extent of hypercalcemia appears more closely related to the defective secretory regulation than to increased parenchymal cell mass [59].
7.2.2 Hyperparathyroidism
Hyperparathryroidism has been diagnosed with increasing frequency in recent years due to awareness of the disease and to the laboratory advancement that allowed for routine chemistry screening. The condition is characterized by excess secretion of parathyroid hormone. The resulting biochemical changes, including increased levels of serum calcium and increased urinary excretion of calcium, may result in calcium wastage, nephrocalcinosis, urolithiasis, bone disease, and neuropsychiatric disturbances. Hyperparathyroidism may occur as a primary, secondary, or tertiary disease. It can also occur as eutopic and ectopic disease. In addition, it may have a familial origin, as in multiple endocrine neoplasia (MEN).
7.2.2.1 Primary Hyperparathyroidism
Primary hyperparathyroidism occurs due to neoplastic or hyperplastic parathyroid glands or when nonparathyroid tumors such as bronchogenic or renal cell carcinomas secrete ectopically parathyroid hormone or a biologically similar product. The incidence in the United States has been estimated at approximately 27.7 cases per 100,000 population per year [60]. The condition is more prevalent in females than males by a ratio of 3 to1. More than 80 % of patients with primary hyperparathyroidism have a solitary adenoma. Hyperplasia—predominantly of chief cells—occurs in less than 20 % of patients. Parathyroid carcinoma is the cause in less than 1 % of patients, and very rarely the condition is due to ectopic secretion of parathyroid hormone [61, 62].
Primary hyperparathyroidism occurs as part of MEN. MEN is a hereditary syndrome that involves hyperfunctioning of two or more endocrine organs. Primary hyperparathyroidism, pancreatic endocrine tumors, and anterior pituitary gland neoplasms characterize type 1 MEN. MEN 2A is defined by medullary thyroid carcinoma, pheochromocytoma (about 50 %), and hyperparathyroidism caused by parathyroid gland hyperplasia (about 20 %). MEN 2B is defined by medullary thyroid tumor and pheochromocytoma. Both MEN 1 and MEN 2 are inherited autosomal dominant cancer syndromes. The gene responsible for MEN 1 is a tumor suppressor gene located on chromosome 11.
7.2.2.2 Secondary Hyperparathyroidism
Secondary hyperparathyroidism occurs when there is a condition causing chronic hypocalcemia such as chronic renal failure, malabsorption syndromes, dietary rickets, and ingestion of drugs such as phenytoin, phenobarbital, and laxatives, which decrease intestinal absorption of calcium. Secondary hyperparathyroidism is simply a compensatory hyperplasia in response to hypocalcemia. In this condition, reduced renal production of 1,25-dihydroxyvitamin D3 (active metabolite of vitamin D) leads to decreased intestinal absorption of calcium, resulting in hypocalcemia. Tubular failure to excrete phosphate results in hyperphosphatemia. Hypocalcemia along with hyperphosphatemia is compensated for by hyperplasia of the parathyroids to overproduce PTH.
7.2.2.3 Tertiary Hyperparathyroidism
Tertiary hyperparathyroidism describes the condition of patients who develop hypercalcemia following long-standing secondary hyperparathyroidism due to the development of autonomous parathyroid hyperplasia, which may not regress after correction of the underlying condition, as with renal transplantation.
7.2.2.4 Eutopic Parathyroid Disease
Parathyroid disease with typical location of glands (eutopic) represents 80–90 % of all cases. There is a relatively fixed location for the superior parathyroids and are found close to the dorsal aspect of the upper thyroid [53, 54]. On the other hand, inferior parathyroids have a more widespread distribution, which is closely related to the migration of the thymus. Inferior parathyroids are mostly located inferior, posterior, or lateral to the lower thyroid [53]. They may be very close to the thyroid and may be covered by or attached to the thyroid capsule and are sometimes adjacent to or surrounded by remnant thymic tissue. Interestingly, the parathyroid glands demonstrate a remarkably constant symmetry, which is helpful in the surgical exploration of eutopic disease [54].
7.2.2.5 Ectopic Parathyroid Disease
Superior parathyroid adenoma may have an abnormal supero-posterior mediastinal position, such as a retropharyngeal, retroesophageal, or paraesophageal site or the tracheoesophageal groove. The frequency of ectopia (up to 39 %) is similar for the right and left superior parathyroids [66]. Intrathyroid superior parathyroid adenomas are rare.
The more common ectopic inferior parathyroids are a well-established entity responsible for 10–13 % of all cases of hyperparathyroidism [66]. Ectopic tissue can occur from the angle of the mandible to the mediastinum according to the developmental and migratory aberrations. These sites include the mediastinum, thymus, aortopulmonary window, carotid bifurcation and rarely thyroid, carotid sheath, vagus nerve, retroesophageal region, thyrothymic ligament, and pericardium [66, 67].
7.2.3 Parathyroid Adenoma
Parathyroid adenoma is a benign tumor that is usually solitary, although multiple adenomas are found in a low percentage. The tumor in general is more common in women and varies in weight from less than 100 mg to more than 100 g. The most commonly found adenomas, however, weigh 300 mg to 1 g. The size was found to correlate to the degree of hypercalcemia.
Microscopically, the vast majority of typical adenomas are formed predominantly of chief cells, although a mixture of oxyphil cells and transitional oxyphil cells is also common. Adenomas formed of water-clear cells are very rare. A rim of parathyroid tissue is usually present outside the capsule of the adenoma and can serve to distinguish it from parathyroid carcinoma. The chief cells in adenomas are usually enlarged, and their nuclei are larger and more variable in size than in normal chief cells. Nuclear pleomorphism may be prominent; this is not considered a sign of malignancy but a criterion for discriminating adenoma from hyperplasia, which lacks this feature. The following variants (Table 7.4) of parathyroid adenoma may be recognized.
Type | Major features | Imaging |
---|---|---|
Solitary adenoma | Found in 80–85 % of patients with primary hyperparathyroidism | 99mTc-MIBI with high sensitivity |
Composed of chief cells or mixture of chief, oxyphil, or transitional oxyphil cells | ||
Double/multiple | Occurs in up to 12 % of cases of primary hyperparathyroidism | Detection with any imaging modality is not reliable |
Bilateral in 55–88 % of cases | 99m Tc-MIBI has a sensitivity of less than 37 % | |
More prominent symptoms and higher parathyroid hormone and alkaline phosphatase levels than with a solitary adenoma or hyperplasia | ||
Cystic adenoma | Central necrosis or cystic degeneration of adenomas | May not be visualized on 99mTc-MIBI studies |
Accounts for less than 9 % of all parathyroid adenomas | ||
Frequently associated with hyperparathyroidism | ||
Lipoadenoma | Composed of hyperfunctioning parathyroid tissue and fatty stroma | Target-to-background ratio may be low due to the high adipose content |
Oncocytic adenoma | Rare subtype formed of 80–100 % oxyphil cells | 99mTc-MIBI with high sensitivity |
7.2.3.1 Solitary Adenoma
Solitary adenoma is found in 80–85 % of patients with primary hyperparathyroidism [11]. There is no significant predominance in location among the four parathyroids with each responsible for approximately 25 % of all solitary adenomas [66]. The remaining tumor-free parathyroid glands associated with single adenomas usually have lower weight and parenchymal cell mass than the average normal glands and show signs of secretory inactivity on electron microscopy [58].
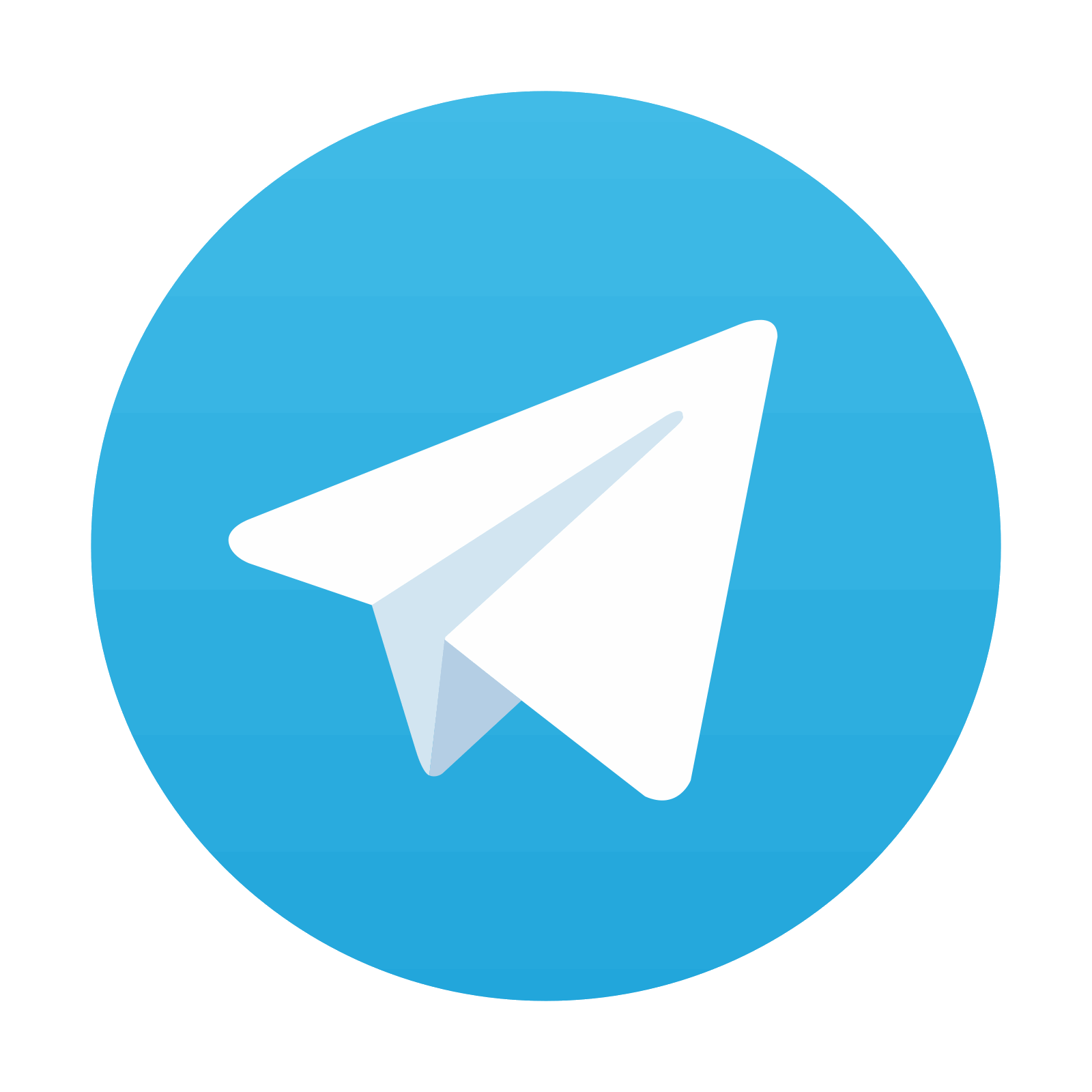
Stay updated, free articles. Join our Telegram channel
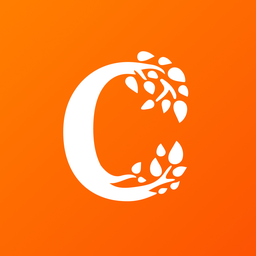
Full access? Get Clinical Tree
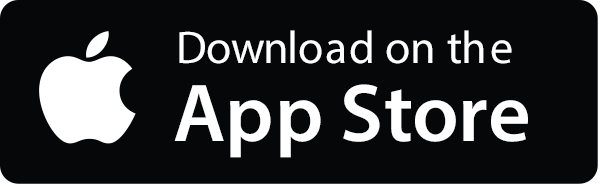
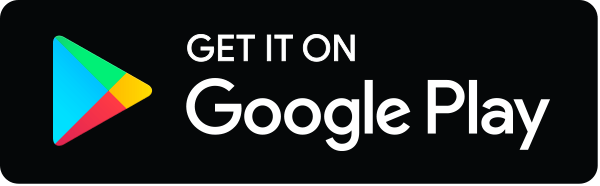
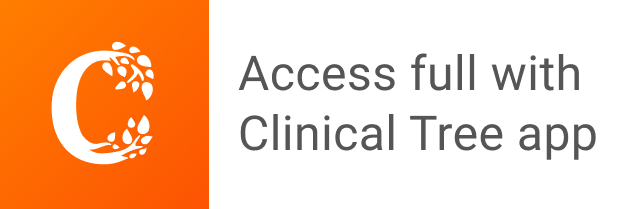