(1)

(2)
Evaluations are usually personalized by using the implemented tools of kinetic models, several phantoms, multiregional organs, and organ mass adjustments.
More recently, methods accounting for the actual morphology and inhomogeneous activity have also been applied to PRRT. In order to step over the mean dose parameter, the voxel dosimetry is being increasingly implemented to derive dose distribution maps and Dose Volume Histograms (DVH); Monte Carlo simulations associated to SPECT and CT images are still very (too) demanding for being of standard clinical use. In any case, the heading is toward a real patient-specific dosimetry—it is just a matter of time. Figure 1 delineates the progression of dosimetry from 2D to 3D standard dose to 3D dose distribution evaluations.
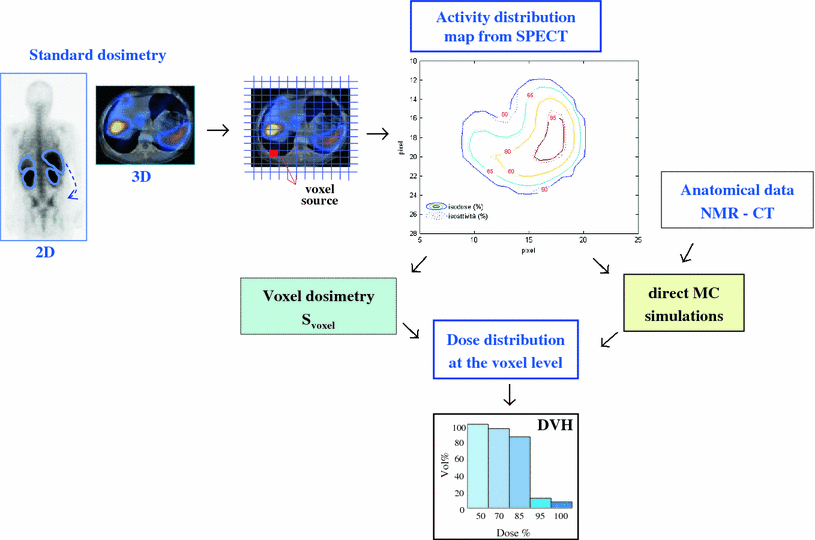
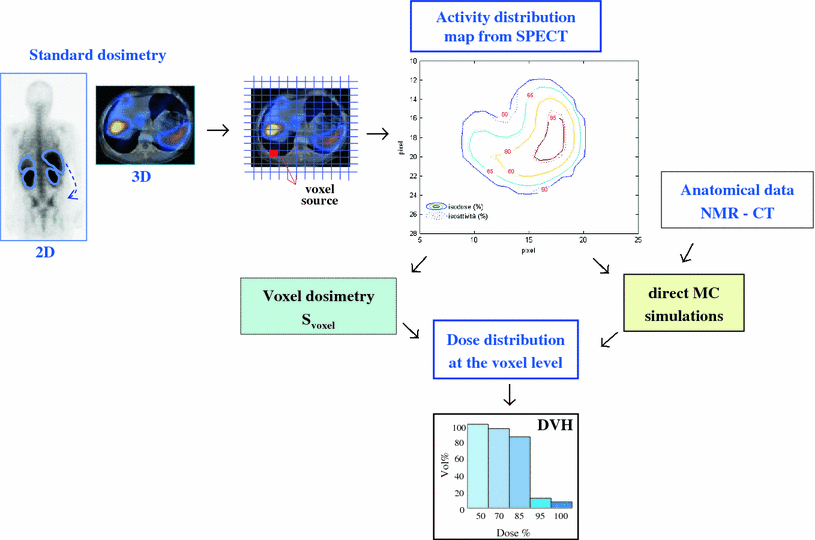
Fig. 1
Dosimetry from 2D and 3D standard methods to 3D dose distribution evaluations. The 3D activity distribution map can be used as input for a voxel dosimetry method (MIRD) or for a direct Monte Carlo simulation with the inclusion of anatomical data from CT or NMR
3.1 Data Collection
Any dosimetry evaluation requires patient-specific data to assess the pharmacokinetics and the biokinetics of the radiopharmaceutical. With respect to the time schedule for data collection, it is essential to collect enough data to describe any uptaking and washout phase (at least 2–3 points for each phase). The graph included in ( Fig. 2) show that too poor information may easily lead to mioseastimates and that the altest time points are very important for a proper curve fitting and estimation of the area under the curve. The results reported for 90Y and 177Lu-peptide confirm a rapid blood clearance and activity elimination from the body, mainly through the kidneys (typically: < 1% of the activity in the blood 1 h post injection (p.i.); ~70–80 % of the activity excreted 1 day p.i.). Scintigraphic images show a significant variation of the uptake in normal organs—especially the spleen, the kidneys, and the liver—within the first day. Typically, all time-activity curves follow a two-exponential trend. Withdrawal of blood samples need to be intensified within the first hours and then distributed in the following 2–3 days. Scintigraphic acquisitions (possibly 5–6) would be appropriate up to at least 3 days p.i.
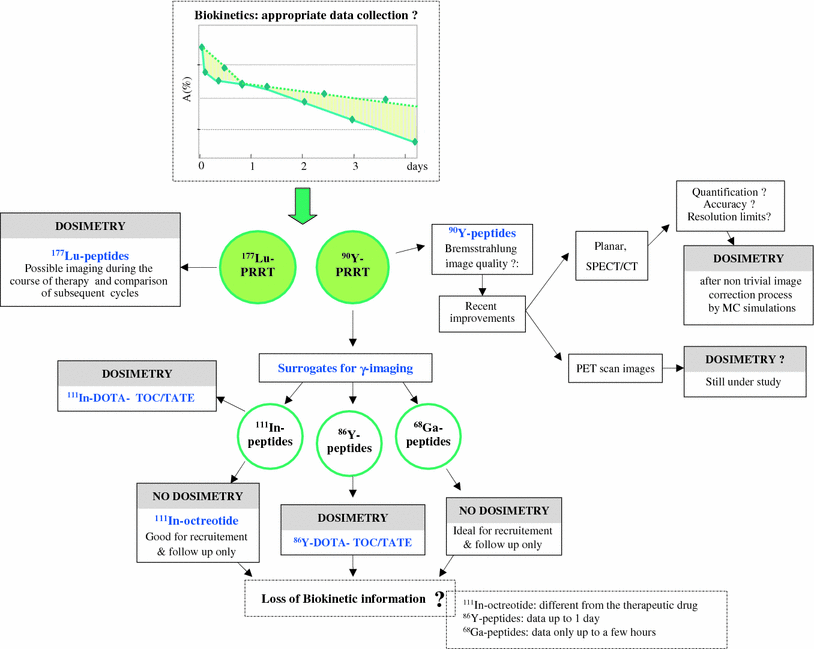
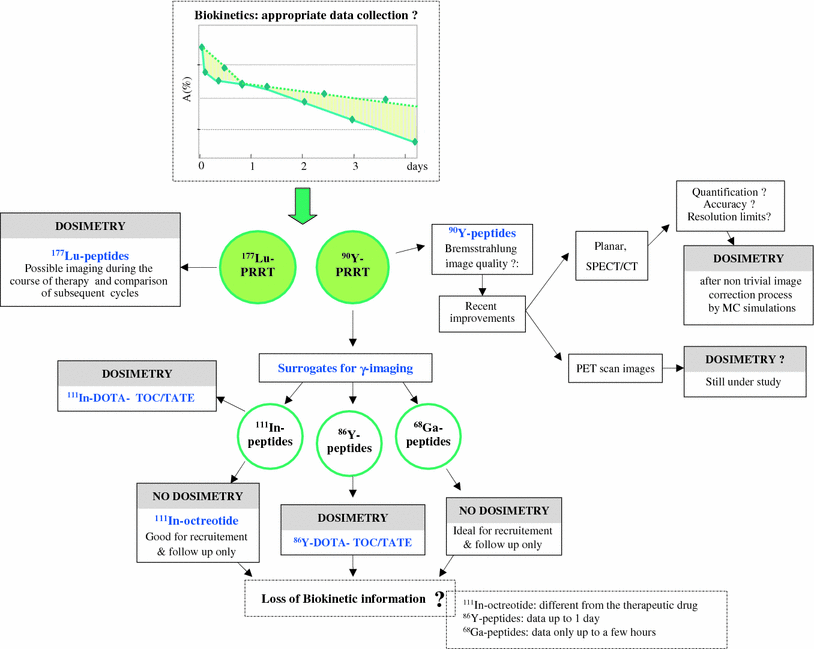
Fig. 2
The possible methods pre- and post-therapeutic imaging and adequacy of the radiotracers for dosimetry purposes. The graph included shows the need of enough data points to study the biokinetics for proper fitting curves and estimation of the area under the curves
As regards the kind of imaging, the choice remains hold to the dosimetry method adoptable. Hybrid 2D and 3D acquisitions might represent a good compromise. Planar views can satisfactorily provide the kinetic trend, while SPECT, possibly SPECT/CT, could assess the 3D activity distribution for the body area relative to the critical organs and tumors. In any case, it is important to adequately elaborate images including attenuation, scatter, and background corrections. Specific procedures are referred to the literature (Dewaraja et al. 2012; Garkavij et al. 2010; Sandström et al. 2010; Sjögreen et al. 2005; Siegel et al. 1999).
3.2 Imaging for Dosimetry
Data collection for dosimetry purposes in PRRT poses the question of considering appropriate radiocompounds able to simulate the biokinetics of the therapeutical agent. The physical properties of 177Lu allow to directly perform dosimetry with 177Lu-peptides before or during therapy. On the contrary, difficulties arise for 90Y-peptides due to the lack of γ emission. Besides difficulties in the Bremsstrahlung image setup for acceptable quality, quantification is far from being confident and effortless. The use of surrogates represents a reasonable solution. Few considerations should guide the choice among the variety of radiolabeled somatostatin analogs which offer fair imaging properties (such as 111In-octreotide, 111In-DOTATOC, 111In- DOTATATE, 86Y-peptides, and 68Ga-peptides …), being different their diagnostic sensitivity/specificity and biokinetics in normal organs (Cremonesi et al. 2010). Clear distinction has to be done between diagnostic and simulation properties, i.e., the asset for patients’ recruitment and follow-up or for the ability to represent metabolic behavior of the therapeutical complex. In particular, 111In-octreotide is limited as a surrogate of 90Y-DOTATOC or 90Y-DOTATATE, because of the different peptide biodistribution. A more suitable alternative uses the same therapeutical compound labeled with 111In, although in principle different influence from 111In versus 90Y on the molecule behavior might not be excluded. 86Y-peptides totally preserve the chemical structure, but arise practical and technical problems: 86Y is costly and scarcely available, its T1/2,phys (14.7 h) limits at 24–40 h the acquisition time, and interference from high energy γ-rays imposes nontrivial image corrections (Pentlow et al. 2000). 68Ga-peptides are not appropriate, despite the diagnostic superiority, as the too short T1/2,phys (68 min) would not allow to collect information for more than few hours. Advantages and pitfalls are in all these surrogates. It has to be remarked that the possibility of Bremsstrahlung image quantification has been seriously re-evaluated. Recent studies have shown the relevant results derivable from the implementation of complex calibration procedures and Monte Carlo simulations to correct for scatter and detector response (Walrand et al. 2011; Minarik et al. 2008, 2009). 90Y imaging is thus promising and might become the future standard approach. The possibilities here described for pre- and post-therapeutic imaging and their adequacy for dosimetry are schematically presented in Fig. 2.
3.3 Dose Estimates
Figure 3 summarizes the principal estimates of average absorbed doses reported in the literature for 90Y-DOTATOC (Fig. 3a) and for 177Lu-DOTATATE (Fig. 3b) (Cremonesi et al. 2006; Forrer et al. 2004, 2009; Forster et al. 2001; Garkavij et al. 2010; Helisch et al. 2004; Hindorf et al. 2007; Jamar et al. 2003; Kwekkeboom et al. 1999, 2001; Rodrigues et al. 2006; Wehrmann et al. 2007). The median values among patients are shown (the variability within each group, always quite high, is not reported for simplicity). It is to be observed that the highest irradiation is usually received by the spleen, while the kidneys are the critical organs for both 90Y and 177Lu labeling radionuclides. After first clinical trials, the need for lowering the kidney uptake led to consider the potential of amino acids as renal protective agents. Specific dosimetric studies addressed the efficacy of different molecules, amounts and infusion modalities, the preferable of which have been subsequently applied in clinical practice (Bodei et al. 2009; Kwekkeboom et al. 2008; Rolleman et al. 2010; van Essen et al. 2009). Thus, older data report higher kidney doses, while the more recent are lower (by 25 % up to 65 % (Bodei et al. 2003, 2008; Cremonesi et al. 2006; Rolleman et al. 2010), depending on the protection type and on the single patient metabolism). Nonetheless, especially with 90Y-peptides, the dose to the kidneys remains alarming, being the dose threshold for radiation nephropathy possibly reached (Bodei et al. 2008; Cassady 1995; Cremonesi et al. 2010). The need of accuracy on renal evaluations has laid aside the methods based on uniform activity distribution and standard kidney mass. A multi-region MIRD model for a sub-organ kidney dosimetry and dose rate effects has been proposed (Bouchet et al. 2003) and included in available software (Stabin et al. 2005). Dose estimates can be further improved by including the activity distribution in the renal cortex and medulla, as derived from SPECT and PET images or even extrapolating information from published results regarding autoradiography analysis (Konijnenberg et al. 2007; Wessels et al. 2008).
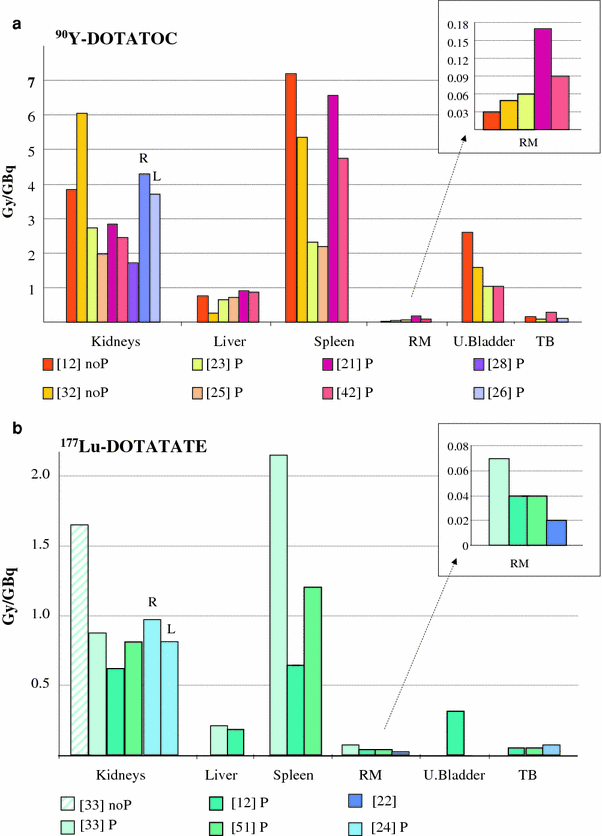
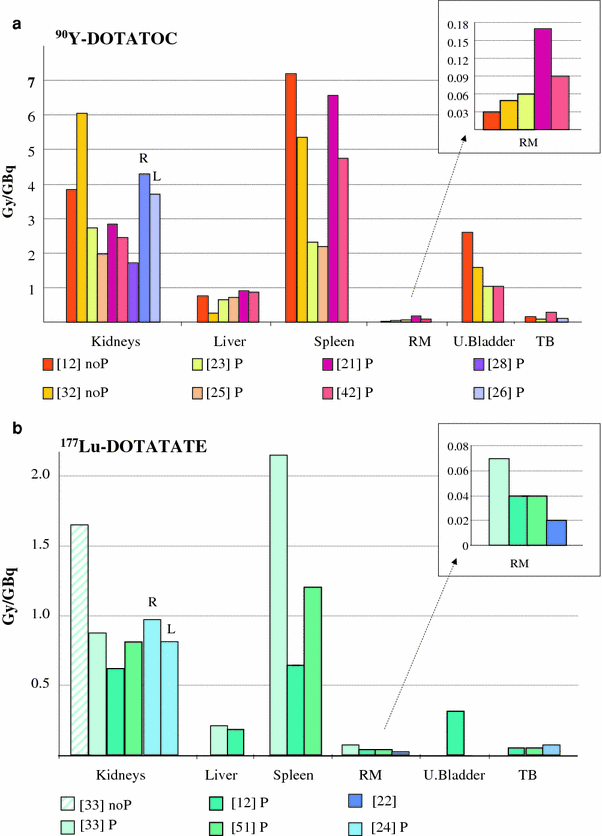
Fig. 3
Principal estimates of average absorbed doses per unit activity reported in the literature for 90Y-DOTATOC (a) and for 177Lu-DOTATATE (b) (Cremonesi et al. 2006; Forrer et al. 2004, 2009; Forster et al. 2001; Garkavij et al. 2010; Helisch et al. 2004; Hindorf et al. 2007; Jamar et al. 2003; Kwekkeboom et al. 1999, 2001; Rodrigues et al. 2006; Wehrmann et al. 2007). The legends specify the reference number as respect to the data provided. The evaluation obtained with or without the administration of renal protectors is also specified (noP no protection, P protection). Bars refer to median values among patients; variability among patients of each group is not reported for simplicity. The estimates for the red marrow are further zoomed (included in the frame on the right side) to allow comparisons. RM red marrow, TB total Body, U. bladder urinary bladder
The bone marrow irradiation related to a therapeutical administration should not suggest special worry of hematological toxicity. In most cases, images do not document any specific uptake—with the exception of some patients studied with 86Y-DOTATOC, for which however the assignment of activity to bone structures might be related to high energy γ-ray interference. A publication (Forrer et al. 2009) focused on the bone marrow dosimetry in PRRT reported no significant binding of 177Lu-DOTATATE to marrow stem cells and analog activity concentration [A] in marrow aspirates and in the blood. The adequacy of the hypothesis assumed in previous studies, easily deriving the number of decays in the red marrow (NDRM) from the integral activity of the blood, has thus been confirmed (see following equations):
![$$ \left[ {A} \right]_{\text{blood}} { = }\left[ {A}
\right]_{ \text{RM}} \to \left[ \widetilde{A} \right]_{\text{blood}}
{ = }\left[ \widetilde{A} \right]_{\text{RM}} \to
{\text{ND}}_{\text{blood}} / {m}_{\text{blood}}
{\text{ = ND}}_{\text{RM}} / {m}_{\text{RM}} , \text{i.e.}
{\text{ND}}_{\text{RM}} = {\text{ ND}}_{\text{blood}} \times
{m}_{\text{RM}} /{m}_{\text{blood}} $$](/wp-content/uploads/2016/09/A174_2012_711_Chapter_Equ3.gif)
where m blood and m RM are the individual blood and RM masses.
![$$ \left[ {A} \right]_{\text{blood}} { = }\left[ {A}
\right]_{ \text{RM}} \to \left[ \widetilde{A} \right]_{\text{blood}}
{ = }\left[ \widetilde{A} \right]_{\text{RM}} \to
{\text{ND}}_{\text{blood}} / {m}_{\text{blood}}
{\text{ = ND}}_{\text{RM}} / {m}_{\text{RM}} , \text{i.e.}
{\text{ND}}_{\text{RM}} = {\text{ ND}}_{\text{blood}} \times
{m}_{\text{RM}} /{m}_{\text{blood}} $$](/wp-content/uploads/2016/09/A174_2012_711_Chapter_Equ3.gif)
(3)
Data from the literature reported red marrow doses per single cycle well below 1 Gy (with common therapy schemes), in spite of the method used—the blood-based or the imaging-based one (Bodei et al. 2004, 2008, 2009; Cremonesi et al. 2010; Forrer et al. 2009; Helisch et al. 2004; Wehrmann et al. 2007).
3.4 90Y- Versus 177Lu-Peptides
The visual comparison of the histograms in Fig. 3a, b highlights that the absorbed doses per unit activity are much higher for 90Y-peptides than those for 177Lu-peptides, with about a same dose ratio for a same activity. This is generally true despite the peptide considered, especially for non target up-taking organs, because of the major impact of the physical properties on the dose—i.e., energy release and T 1/2,phys.
In a specific study extrapolating dosimetric evaluations from 177Lu-DOTATATE to 90Y-DOTATATE in the same patients, a ND ratio (90Y/177Lu) of about 0.6–0.8 (Fig. 4a) and a dose ratio of about 3.5–5.5 (Fig. 4b) has been found in almost all normal organs. On the contrary, much wider variability of the dose ratio was observed in tumors, ranging from 2 to 4.5, with the tendency of more favorable tumor/kidney ratios with 177Lu for smaller lesions, while 90Y for bigger ones (Cremonesi et al. 2006, 2010). The conclusion was that the tumor/kidney dose ratio is not always in favor of the same radionuclide, and that the benefit/risk balance should be established for each patient. Also, too simple deductions to identify a preferable radionuclide should not be made. The influence of different peptides, target, and non-target tissue receptor expression, peptide-radionuclide associations, individual metabolic behavior, and tumor characteristics may lead to unexpected consequences. Some issues have been clarified, others need further analysis.
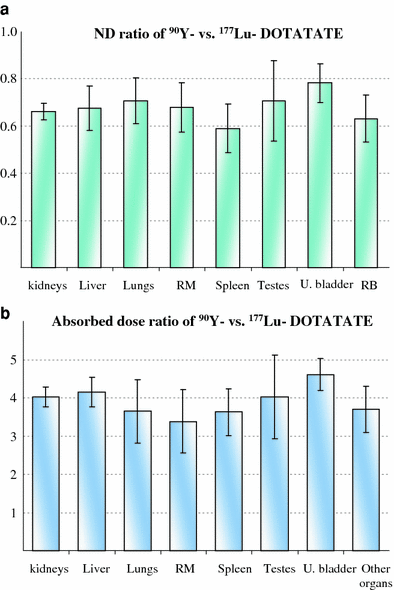
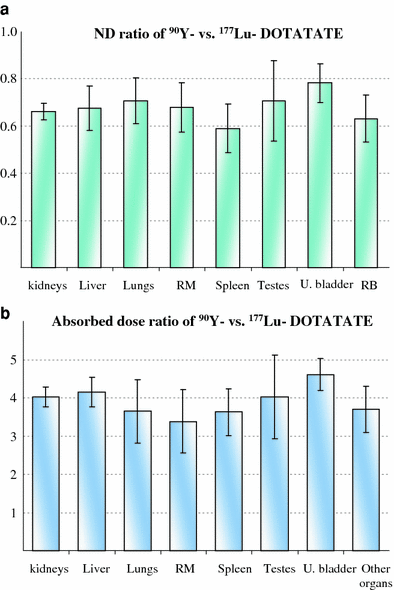
Fig. 4
Comparison of 177Lu-DOTATATE and 90Y-DOTATATE dosimetric evaluations in the same patients. a shows the ND ratio (90Y/177Lu) for the principal source non-target organs identified. b shows the absorbed dose ratio (90Y/177Lu) per unit activity
Although in a low number of cases, the above results are consistent with preclinical results (de Jong et al. 2005), and find explanation in the dosimetry models. The curve in Fig. 5 describes the mass dependence of the DF (dose conversion factor) ratio for 177Lu versus 90Y for hypothetical tumors of spherical shape and uniform uptake. As the mass decreases, 90Y releases a greater part of the energy outside the sphere, in contrast with 177Lu, and the ND ratio dramatically increases in favor of 177Lu; as the mass increases and approaches the particle range of 90Y, the curve shows a slight slope trend. This clearly supports the advantage of 177Lu for small lesions and of 90Y for big ones. Once leaving these simplified situations for the real ones, many other factors which might influence the response come into sight.
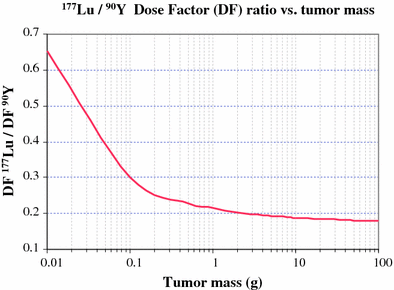
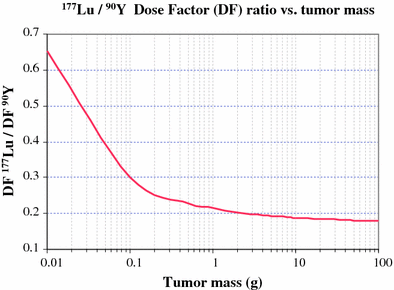
Fig. 5
Mass dependence of the DF (dose conversion factor) ratio for 177Lu versus 90Y for hypothetical tumors of spherical shape and uniform uptake. As the mass decreases, 90Y releases a greater part of the energy outside the sphere, in contrast with 177Lu, and the ND ratio dramatically increases in favor of 177Lu; as the mass increases, and approaches the particle range of 90Y, the curve shows a slight slope trend
Tumor inhomogeneity figures as recurrent trait; the cross-fire effect of 90Y might be a trump card to be played against unfavorable activity distribution, for a superior radiation burden (Botta et al. 2008; Cremonesi et al. 2010; O’Donoghue 1999). In addition, individual differences in tumor mass, location, receptor density, vascularization, radiosensitivity, peptide kinetics, cell internalization, adjacent tissues, etc…, should be charily pondered to guide the choice of the most suitable radionuclide (or radiopharmaceutical). Different radionuclide properties offer different benefits, so combinations of 177Lu and 90Y-peptides have been correctly proposed (de Jong et al. 2005; Kunikowska et al. 2009).
The impact of the choice of the radionuclide cannot be restricted to the tumor; it must be evaluated also in the kidneys, for which a uniform uptake represents just a first approximation. Relevant differences and new questions emerge when switching from an even activity in the whole organ to a non uniform activity distributed in renal sub-regions. As shown in published dose volume histograms (Wessels et al. 2008), assuming an uniform activity distribution, the choice of 177Lu or of 90Y would imply only slight differences, limited to the interfaces (organ edge)—as expected (see also Fig. 5b for typical kidney mass). On the contrary, the activity inhomogeneity enhances the higher dose inhomogeneity for 177Lu- as compared to 90Y-derivatives (as a consequence of cross-over effect). These differences between the two radionuclides suggest possible renal burden, which may depend on the macro- and/or microscopic activity distribution. Present knowledge cannot conclusively identify 177Lu or 90Y as safer radionuclide for kidneys. Studies on this topic are ongoing (Cremonesi et al. 2009; Svensson et al. 2009).
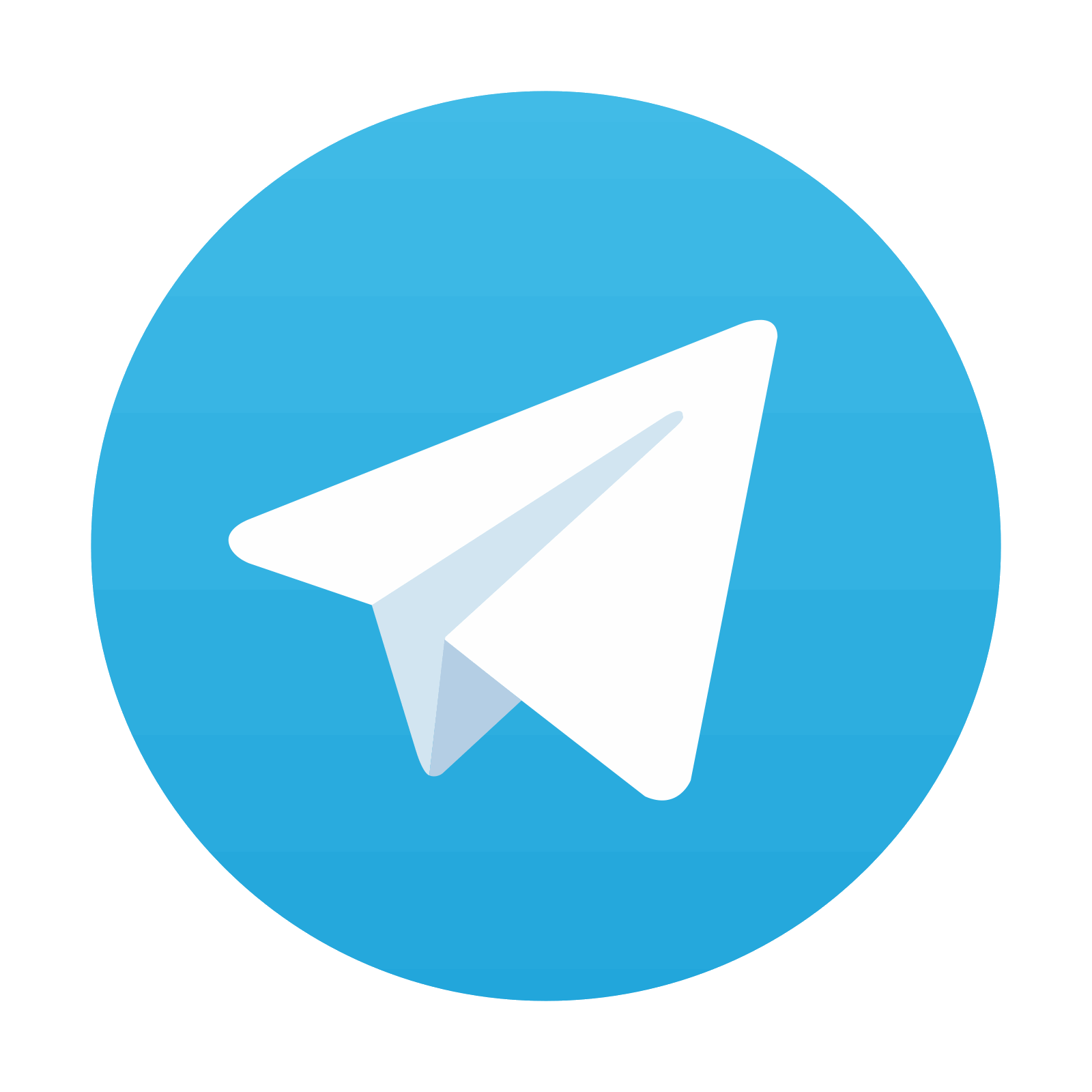
Stay updated, free articles. Join our Telegram channel
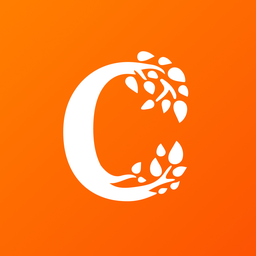
Full access? Get Clinical Tree
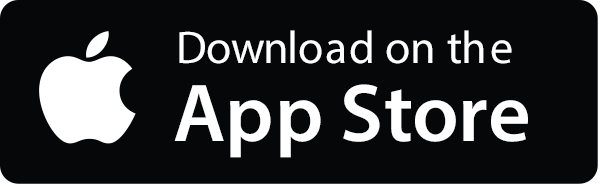
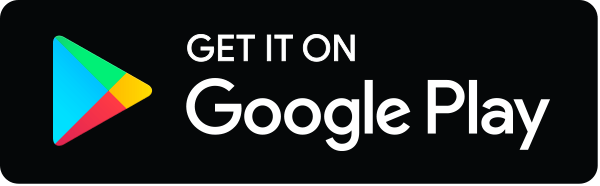