Here D β is the beta dose in rad, C is the concentration of the nuclide in the tissue in microcuries per gram, E β is the mean energy emitted per decay of the nuclide, and T is the effective half-life of the nuclide in the tissue. Although proposed more than 50 years ago and superseded by other more complex methods, some still use this simple formula effectively for thyroid dose calculations. Radiopharmaceutical dose estimates for standardized applications with multiple source and target organs may be calculated using the methodology developed by the radiation dose assessment resource (RADAR) Task Group of the Society of Nuclear Medicine (Stabin and Siegel 2003). This method uses a simple equation for internal dose to nuclear medicine patients and radiation workers, as well as external dose in any situation:
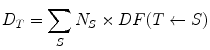
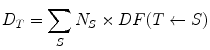
(1)
Here D T is the dose to target region T, N S is the number of nuclear transitions that occur in source region S, and DF(T ← S) is the ‘dose factor’ for source region S irradiating target region T. This method reflects all of the concepts employed in dose methodologies proposed by the international commission on radiological protection (ICRP) (International Commission on Radiological Protection 1979) and medical internal radiation dose (MIRD) (Bolch et al. 2009), in an understandable and easily used method.
To perform a simple dose calculation, one needs to calculate the value N S (which is simply the integral of the time-activity curve for a source organ) and then apply the appropriate dose factors, which contain both the decay data and absorbed fractions (AFs) for internal organ self- and cross-irradiation. Methods for quantifying image data for dose calculations, with example calculations, are described in detail in the textbook on nuclear medicine dose calculations by Stabin (2008). The AFs are derived generally using Monte Carlo simulation of radiation transport in models of the body and its internal structures (organs, tumors, etc.). Standard models of the body are defined for adults, children, pregnant women, and various other structures. The standard models for adults and children used for many decades were described by Cristy and Eckerman (1987); new, more realistic models, based on models employing non uniform rational B-splines (NURBS) have now been defined by the RADAR group (Stabin et al. 2008) (Fig. 1).
The simple dose formula above is summed over all important source organs to give the total dose to each target organ, with the source organs chosen depending on the biokinetic behavior of each radiopharmaceutical. The RADAR dose calculation method has been implemented in the OLINDA/EXM personal computer code (Stabin et al. 2005). Table 1 shows sample output from the OLINDA/EXM code for administration of 99mTc diagnostic bone agents to an adult (International Commission on Radiological Protection 2009).
Table 1
Radiation dose estimates for 99mTc-labeled phosphates and phosphonates
Estimated absorbed dose | ||
---|---|---|
Target organ | mGy/MBq | rad/mCi |
Adrenals | 2.10E-03 | 7.77E-03 |
Bladder | 4.80E-02 | 1.78E-01 |
Bone surfaces | 6.30E-02 | 2.33E-01 |
Brain | 1.70E-03 | 6.29E-03 |
Breasts | 7.10E-04 | 2.63E-03 |
Gall bladder | 1.40E-03 | 5.18E-03 |
GI-tract: | ||
Stomach | 1.20E-03 | 4.44E-03 |
Small intestine | 2.30E-03 | 8.51E-03 |
Colon | 2.70E-03 | 9.99E-03 |
Upper large intestine | 1.90E-03 | 7.03E-03 |
Lower large intestine | 3.80E-03 | 1.41E-02 |
Heart | 1.20E-03 | 4.44E-03 |
Kidneys | 7.30E-03 | 2.70E-02 |
Liver | 1.20E-03 | 4.44E-03 |
Lungs | 1.30E-03 | 4.81E-03 |
Muscles | 1.90E-03 | 7.03E-03 |
Esophagus | 1.00E-03 | 3.70E-03 |
Ovaries | 3.60E-03 | 1.33E-02 |
Pancreas | 1.60E-03 | 5.92E-03 |
Red marrow | 9.20E-03 | 3.40E-02 |
Skin | 1.00E-03 | 3.70E-03 |
Spleen | 1.40E-03 | 5.18E-03 |
Testes | 2.40E-03 | 8.88E-03 |
Thymus | 1.00E-03 | 3.70E-03 |
Thyroid | 1.30E-03 | 4.81E-03 |
Uterus | 6.30E-03 | 2.33E-02 |
Remaining organs | 1.90E-03 | 7.03E-03 |
Effective dose (mSv/MBq) | 5.70E-03 | 2.11E-02 |
The 90Y-labeled monoclonal antibody Zevalin (ibritumomab tiuxetan) was approved for use with no requirement for calculation of radiation doses to individual subjects, only verification that the pre-therapy biological distribution does not appear abnormal. The similar 131I-labeled product, Bexxar (tositumomab), however, is administered with a simplified patient-individualized dose calculation. Image data are quantified at three time points observed over several days, the area under the time-activity curve (N S ) is estimated graphically, and the average dose to an ellipsoid whose mass is similar that of the patient’s whole body is used as a surrogate for dose to red marrow, and is limited to 750 mGy.
More sophisticated dose calculations have been performed using image data to perform dose calculations, including the 3D-ID and 3D-RD codes (Kolbert et al. 1997; Prideaux et al. 2007), the SIMDOS code from the University of Lund (Dewaraja et al. 2005), the RTDS code at the City of Hope Medical Center (Liu et al. 1999), the RMDP code from the Royal Marsden Hospital (Guy et al. 2003), the DOSE3D code (Clairand et al. 1999) and PEREGRINE code (Lehmann et al. 2005). In these applications, 3-D anatomical data, from computed tomography (CT) or magnetic resonance (MR) images, are fused with 3-D activity distributions, from single photon emission computed tomography (SPECT) images taken from the individual patient over time.
This level of analysis is comparable to that applied in external beam therapy or brachytherapy dose calculations, but, unlike OLINDA/EXM, software for this approach has not yet become widely available (Fig. 2).
4 Treatment planning systems for TRT
Variations in treatment planning methodologies for external beam radiotherapy (EBRT) and brachytherapy tend to be minor and are well established with clinical studies. Similarly, the majority of protocols for chemotherapy regimens are also formulated via clinical trials. In contrast, TRT is possibly the only cancer treatment modality that does not have established systems for planning treatment.
Both TRT and EBRT are based on the delivery of radiation to malignant tissues whilst minimising the absorbed doses delivered to organs-at-risk. Whilst EBRT can be planned to deliver an absorbed dose distribution, tailored to an individual, to within 97–107 % of a prescribed tolerance dose [ICRU 50, ICRU 60], this is only possible within TRT if the radiopharmaceutical is administered directly into a tumor or resected cavity under the assumption that activity will not leak beyond the target volume. For this treatment only a mean absorbed dose can be reliably predicted due to varying diffusion patterns (Akabani et al. 2000; Flux et al. 1997). In the majority of cases administrations are oral, intra-venous or intra-arterial and the pattern of uptake and retention following administration has been shown to vary widely from patient to patient.
Ideally, treatment planning for TRT must adopt approaches used for chemotherapy whilst aiming at a treatment plan that resembles that produced for EBRT.
There are a number of parameters, available to the treating physician, that will directly or indirectly influence the delivery of an absorbed dose distribution. These may be considered separately:
1.
The Radionuclide. An increasing number of radionuclides are becoming available for TRT. Each has distinct characteristics that render it more or less suitable for a given treatment, and possibly for a given patient. Radionuclides may be considered according to their physical half-lives and their emissions, which govern the range of therapeutic impact, the feasibility, and quality of imaging and aspects of radiation protection. Issues to take into consideration when choosing the optimal radionuclide include the degree of heterogeneity of uptake at a multi-cellular scale, as a longer range emitter would be more effective if uptake is not uniform, the requirements for imaging, if any, that will facilitate dosimetry, and the biological half-life in tissues, as, for example, administration of a long-lived radionuclide would entail unwarranted radiation exposure to staff and patients if it was swiftly washed out. In practice, the radionuclide chosen is based on logistics of accessibility, radiation protection, and licensing, as well as its labeling properties. Issues of the ideal radionuclide are still far from resolved as can be seen from ongoing studies. For example, bone metastases are currently being treated with Re-186, Re-188, Sr-89, Sm-153, and more recently the alpha emitter Ra-223 (O’Sullivan et al. 2006).
2.
The Pharmaceutical. A range of cellular targets are available within cancers treated with TRT which has led to a variety of vectors being developed. In many cases the treating physician is presented with a choice of radiopharmaceuticals that operate with different uptake mechanisms. For example, neuroendocrine tumors can be targeted by meta-iodo-benzyl guanidine (mIBG) which is internalized via the noradrenaline receptor (and can be radiolableled with I-123 for imaging and by I-131 for imaging or therapy (Monsieurs et al. 2002, 2009) and by radiolabeled peptides that target somatostatin receptors over-expressed on tumor cell membranes (Cremonesi et al. 2006). Ideally, patients would be treated according to dosimetric calculations performed on tracer scans of each possible agent. Similarly, radioimmunotherapy has been used to treat NHL with a variety of monoclonal antibodies that target different antigens (DeNardo et al. 2001; Wahl 2005; Wiseman et al. 2001).
3.
Level of administration. The activity administered for a TRT treatment is often fixed, although may be based on weight or more rarely body surface area. It is commonly the case that treatments of commercially available radiopharmaceuticals are standardized according to company recommendations (Bodei et al. 2008; Lau et al. 1994), although a wide variation is seen where products are not commercialized. For example, a recent survey in the UK has found that for I-131 NaI treatment of thyroid cancer, ablation activities can range from 1100 to 4500 MBq (Flux et al. 2011). Studies currently underway in the UK and France are randomizing patients between 3000 MBq, which has been a generally accepted standard in Europe, and 1100 MBq, which is more prevalent in the US. It is evident that the level of administration is one of the main parameters that can be determined on a case-by-case basis and that this should be predicated on the hypothesis that a higher level of administration will result in higher absorbed doses to both tumor and to normal tissues, possibly diminishing as saturation occurs.
4.
The frequency of administration. Along with the level of administration, this parameter is also most easily altered in the clinic and is also seen to vary widely. Radiobiological arguments imply that a single large administration can be more beneficial than a series of smaller administrations (O’Donoghue and Sgouros 2000), although the situation is complicated by many factors. For example, tumors can shrink during or following treatment and in the event of a heterogeneous uptake, partial irradiation to the well-vascularised periphery of a large solid tumor may render the hypoxic interior more accessible to a subsequent delivery. A further consideration is that it is often the case that the effect of treatment may not be seen until many months following administration.
5.
Mechanisms allowing increased activities. Lysine, gelofusine, and amifostine have been used to block kidney uptake in peptide receptor radionuclide therapy and potassium iodide has been used for many years to block thyroid uptake during I-131 mIBG treatments (Rolleman et al. 2010; Brans et al. 2002). In principle, such techniques offer the opportunity to administer higher activities safely. A similar effect can be gained by enabling peripheral blood stem cell support or bone marrow support in treatments that may be myeloablative (Gaze et al. 2005).
Taken in isolation, each of these parameters can significantly affect the absorbed doses that may be safely delivered. The combination of any two or more of these parameters leads to a myriad of possible treatment options that can only be examined in a systematic manner by accurate calculation of the absorbed doses in controlled clinical studies. Among the possibilities to be explored are:
1.
The combination of radiopharmaceuticals in a ‘cocktail’ that can utilize the different energies of different radionuclides and different uptake mechanisms(De Jong et al. 2005). For example, treatment of neuroendocrine tumors with the combination of a peptide radiolabeled with the long range emitter Y-90 and I-131 mIBG could significantly improve the therapeutic window as both would target the tumor, although they would not cause excess toxicity as the kidney is the organ-at-risk for PRRT whilst in the case of mIBG therapy this the bone marrow.
2.
The combination of TRT with chemotherapy that can act as a radiosensitiser. This has been utilized in neuroblastoma treatments (Gaze et al. 2005; Buckley et al. 2007; Mastrangelo et al. 1995) with topotecan and cisplatin administered concomitantly with I-131 mIBG, and has been the subject of significant pre-clinical exploration (McCluskey et al. 2005).
3.
The combination of TRT with other modalities. It has, for example, been demonstrated in principle that the planned combination of TRT with IMRT can, as with the case of cocktails of radiopharmaceuticals, deliver higher activities to tumors than is possible with either modality alone, as each causes a different normal tissue toxicity (Bodey et al. 2003).
Despite the difficulties inherent in treatment planning for TRT, there are two main advantages that are not seen in other modalities. Firstly, where dosimetry has been performed, it has been convincingly demonstrated that whilst inter-patient variations in uptake and retention of a radiopharmaceutical can vary from one patient to the next by as much as two orders of magnitude (Flux et al. 2010; Matthay et al. 2001), intra-patient variation tends to be very small (Hindorf et al. 2007). For example, it was shown by Gaze et al. (2005) that pediatrics undergoing I-131 mIBG therapy for neuroblastoma can be treated with a total of 4 Gy whole-body dose by administering the first treatment according to body weight and the second according the whole-body dose resulting from the first treatment. This offers the potential for ‘interactive’ treatment planning whereby activities (and possibly radiopharmaceuticals) are altered during the course of a series of administrations.
The second, and greatest, benefit offered to treatment planning for TRT, is that this is the only cancer treatment modality available that allows indirect visualization of the distribution of delivered absorbed doses via the direct visualization of uptake of the administered agent.
5 The Need for Patient-Individualized Dosimetry
As was stated in 2002 by Siegel et al. (2002):
If one were to approach the radiation oncologist or medical physicist in an external beam therapy program and suggest that all patients with a certain type of cancer should receive the exact same protocol (beam type, energy, beam exposure time, geometry, etc.), the idea would certainly be rejected as not being in the best interests of the patient. Instead, a patient-specific treatment plan would be implemented in which treatment times are varied to deliver the same radiation dose to all patients. Patient-specific calculations of doses delivered to tumors and normal tissues have been routine in external beam radiotherapy and brachytherapy for decades. The routine use of a fixed GBq/kg, GBq/m2, or simply GBq, administration of radionuclides for therapy is equivalent to treating all patients in external beam radiotherapy with the same protocol. Varying the treatment time to result in equal absorbed dose for external beam radiotherapy is equivalent to accounting for the known variation in patients’ uptake and retention half-time of activity of radionuclides to achieve equal tumor absorbed dose for internal-emitter radiotherapy. It has been suggested that fixed activity-administration protocol designs provide little useful information about the variability among patients relative to the normal organ dose than can be tolerated without dose-limiting toxicity compared to radiation dose-driven protocols.
Stabin went so far as to assert (Stabin 2008):
Treating all nuclear medicine patients with a single, uniform method of activity administration amounts to consciously choosing that these patients be treated with a lower standard of care than patients who receive radiation externally for cancer treatments. In all forms of radiotherapy with internal emitters, a patient-individualized dose calculation should be made when possible for all tissues in which a significant uptake of the radiopharmaceutical is observed (including tumors), with dose estimates calculated for these and other important regions (e.g. the bone marrow). Differences in patients’ uptake and clearance rates of radiopharmaceuticals, measured by a minimum of two to three data points, should be catalogued, and published where possible.Stay updated, free articles. Join our Telegram channel
![]()
Full access? Get Clinical Tree
![]()
![]()