CHAPTER 25 Fracture and Hemorrhage
Traumatic brain injury is common in all age groups and is a leading cause of death and disability, especially in young people. The National Center for Injury Prevention and Control estimates that 1.5 million individuals sustain traumatic brain injury in the United States each year. Of these, 50,000 die and 80,000 to 90,000 suffer long-term disability.1 More than 5.3 million Americans currently are disabled by head injury.2 The economic costs exceed $60 billion annually.3 Caring for a victim of severe traumatic brain injury can cost more than a million dollars over a lifetime.
Traumatic brain injury is about twice as common in males as females; African-Americans are affected disproportionately.4 Leading causes of traumatic brain injury are motor vehicle crashes, falls, and firearm use. Use of firearms causes 10% of all traumatic brain injuries but 44% of all deaths, because 90% of all gunshot wounds to the brain are fatal.
CLASSIFICATION OF HEAD INJURY
Traumatic brain injuries may be classified as primary or secondary and subclassified by the severity of injury. Primary brain injuries are those that occur at the time of impact and are a direct result of the traumatic force. Examples include contusions, diffuse axonal injury, intracerebral hematoma, and extra-axial hematomas. Secondary injuries are those that occur later as a consequence of the primary traumatic event and include vasospasm, infarction secondary to vascular compression and brain herniation, anoxic injury, fat embolism, and infection.5 Severity is graded by either the clinical Glasgow Coma Scale (GCS) or the CT-based Marshall score. When they are concordant, both are quite reliable. When they are discordant, additional imaging studies such as MRI should be considered. Clinical outcome is graded by the modified Rankin scale.
Glasgow Coma Scale
The severity of traumatic brain injury is usually classified according to the GCS (Table 25-1).6 This standardized clinical test evaluates three parameters: (1) best eye-opening response, (2) best verbal response, and (3) best motor response. The scale ranges from 3 to 15, with 3 being an unresponsive, flaccid patient and 15 being a fully awake individual. Patients with GCS scores of 13 to 15 are considered to have minor head injuries; those with a score of 9 to 12 have moderate head injury; a score of 5 to 8 indicates severe head injury; and 3 to 4 indicates a very severe head injury.7 The GCS score correlates well with morbidity and mortality.8,9 For example, persistent vegetative state or death occurs in only 6% of patients with a GCS of 11 or higher but in 80% of those with a GCS of 5 or lower. The GCS score at 6 hours after injury predicts outcome more accurately than the immediate GCS score.10 The GCS is less useful for assessing intoxicated trauma victims and infants. Metabolic conditions such as diabetic acidosis or hypoxia may skew the GCS score unfavorably.
Points | |
---|---|
Eye Opening Response | |
Spontaneous—open with blinking at baseline | 4 |
To verbal stimuli, command, speech | 3 |
To pain only (not applied to face) | 2 |
No response | 1 |
Verbal Response | |
Oriented | 5 |
Confused conversation but able to answer questions | 4 |
Inappropriate words | 3 |
Incomprehensible speech | 2 |
No response | 1 |
Motor Response | |
Obeys commands for movement | 6 |
Purposeful movement to painful stimulus | 5 |
Withdraws in response to pain | 4 |
Flexion in response to pain (decorticate posturing) | 3 |
Extension response in response to pain (decerebrate posturing) | 2 |
No response | 1 |
The Marshall Score
The Marshall score utilizes the initial CT scan to assess the severity of diffuse head trauma (Table 25-2).11 This score evaluates the perimesencephalic cisterns, the amount of midline shift, and the presence or absence of one or more surgical masses to assign patients to one of four groups of increasing severity. Individuals classified as having Diffuse Injury I (no visible pathology) have a mortality of 10%. Those with Diffuse Injury IV (midline shift >5 mm and no high- or mixed-density masses >25 mL) have a mortality rate greater than 50%. The Marshall classification allows certain subsets of patients to be directed toward specific types of therapy.12
The Modified Rankin Scale Score
The modified Rankin scale is used primarily to classify the level of disability in stroke patients13 but can also be applied to head trauma victims (Table 25-3).14 The scale ranges from 0 (no symptoms) to 6 (dead). This grading system assesses the functional outcome of the individual and looks at the whole patient in terms of limitations of activity and changes in lifestyle.15
TABLE 25-3 Modified Rankin Scale
Score | Description |
---|---|
0 | No symptoms at all |
1 | No significant disability despite symptoms; able to carry out all usual duties and activities |
2 | Slight disability; unable to carry out all previous activities but able to look after own affairs without assistance |
3 | Moderate disability; requiring some help but able to walk without assistance |
4 | Moderately severe disability; unable to walk without assistance and unable to attend to own bodily needs without assistance |
5 | Severe disability; bedridden, incontinent, and requiring constant nursing care and attention |
6 | Dead |
Data from Bonita R, Beaglehole R. Modification of Rankin Scale: recovery of motor function after stroke. Stroke 1988; 19:1497–1500.
MECHANISMS OF HEAD INJURY
Blunt Head Injury
Most forms of blunt traumatic brain injury are the result of dynamic loading, in which the forces acting on the head occur in less than 200 ms.16 The most common type of dynamic loading is impact loading, in which a blunt object strikes the head or the head strikes an object. Examples would include objects that the head strikes in a motor vehicle accident (windshield, dashboard) or in a fall (ground, wall). With contact, a complex series of events occurs at the site of impact and remote from the point of contact. Directly at the point of impact the skull bends inward. Peripheral to the point of impact the skull bends outward. From the point of impact, shock waves propagate throughout the skull and brain at the speed of sound. The specific type and severity of brain injury depend on the mass and hardness of the object struck and on the velocity at time of impact. As a result of inertial forces, the sudden deceleration of the head causes compressive forces at the site of impact and rarefaction in the tissues on the opposite side of the head.
Thus, the brain is exposed to three different types of strain: compressive, tensile, and shear. Bone, brain, and vascular tissue withstand compressive strain better than tensile strain, especially because brain tissue is essentially incompressible in vivo. Therefore, most injuries to the brain result from tensile and shear strains. Shear strains develop because different tissues like gray matter and white matter have different rates of acceleration/deceleration. Shear strains are especially frequent with rotational acceleration forces. The terms coup and contrecoup have been used for years to describe the type of lesion at the site of impact and opposite the site of impact, respectively. Some investigators eschew these terms as imprecise,5 but they are convenient for descriptive purposes when categorizing the mechanism of a head injury. Compressive phenomena most often cause injury at the point of impact (coup injury), whereas rarefaction phenomena often cause more severe damage at sites distant from the site of impact (contrecoup injury). This concept holds not only for brain contusions but also for some extra-axial lesions, such as subdural hematoma.
Pathophysiology
Blunt and penetrating trauma initially cause mechanical distortion of brain tissue. However, direct primary mechanical disruption of axons and instantaneous cell death is relatively uncommon in traumatic brain injury.16 Instead, these injuries temporarily disrupt the integrity of the cell membrane, setting off a biochemical cascade that leads to secondary injury. Potassium leaks out of the cell. Sodium, calcium, and chloride diffuse in. High levels of intracellular calcium injure the cell and lead to delayed cell death. Additional cell damage is propagated by oxidative free radicals, glutamate,17–19 and inflammatory events.20 Excitatory amino acids, including glutamate and aspartate, are elevated after traumatic brain injury and can induce swelling, vacuolization, and death of neurons as well as astrocytic swelling.21
Scalp and Skull Injury
Scalp Injury
The scalp consists of five layers. From superficial to deep these are the skin, subcutaneous fibrofatty tissue, galea aponeurotica, loose areolar connective tissue, and periosteum.22 Following head trauma, hemorrhage may accumulate in the subcutaneous, subgaleal, and subperiosteal compartments. Subcutaneous and subperiosteal hematomas are much more common in infants and may result from birth trauma. Subgaleal hematomas are the type most frequently seen in adults. Because the subgaleal space lies deep to the frontalis and occipitalis muscles but superficial to the temporalis muscles, subgaleal hemorrhages are often identified on noncontrast CT as crescentic or elliptical areas of high density superficial to the temporalis muscle (Fig. 25-1).
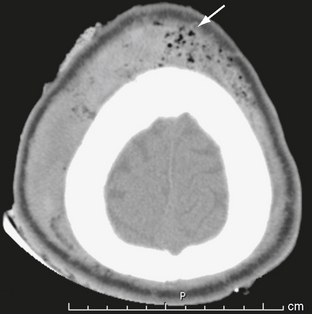
FIGURE 25-1 Scalp hematoma in a 23-year-old man. CT shows large high-density mass in scalp on right. Arrow indicates air in the wound.
Lacerations of the scalp may accompany both penetrating and closed-head trauma. Any lacerations identified should be scrutinized for foreign material, which may be a source of infection (Fig. 25-2). Extensive scalp avulsion, or “degloving,” is sometimes seen in unrestrained passengers ejected from moving vehicles (Fig. 25-3).
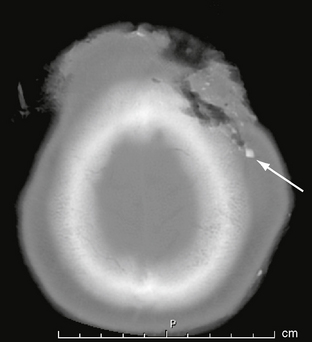
FIGURE 25-2 Scalp and skull laceration in a 30-year-old man. CT demonstrates soft tissue and bone laceration, as well as foreign material (arrow) in the wound.
Skull Fractures
Skull fractures are present in only 25% of fatal head injuries at autopsy.23 Patients with a skull fracture have a higher incidence of intracranial hematoma than those without fracture.14 When a blunt object strikes the head, the local inbending causes a compressive strain on the outer table and a tensile strain on the inner table. Because bone is more susceptible to tensile strain, the fracture begins in the inner table.16 It then propagates along the lines of least resistance. When the skull strikes a broad or flat object, as in a fall, a linear fracture commonly results (Fig. 25-4). Depressed fractures result from more intense forces delivered over a smaller area of impact. Diastatic fractures are linear fractures that intersect or occur along a suture line, causing diastasis of the suture. Reopening of a closed suture is a special form of fracture that may be called sutural diastasis. Basal skull fractures result from blows directly to the occiput or mastoid or from forces transmitted from blows to the face. They also frequently accompany gunshot injury.
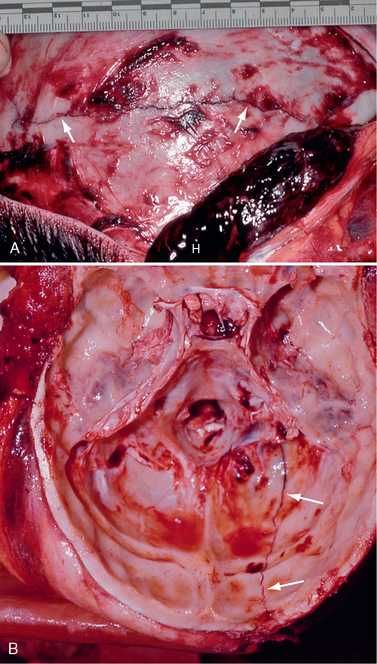
FIGURE 25-4 Linear skull fractures. Postmortem photographs. A, A large temporalis muscle hematoma (H) has been reflected away from the linear fracture line (arrows) that extends across the temporal squama. (See Fig. 25-17 for the internal view of this fracture line as it crosses the grooves for the ascending branches of the middle meningeal artery.) B, Left occipital fracture (arrows) and contrecoup fractures of both orbital roofs secondary to the occipital impact.
(Courtesy of John Deck, MD.)
Noncontrast CT with bone algorithm is the study of choice for imaging all skull fractures (Figs. 25-5 and 25-6). It is important to study the scout image of the CT scan in addition to the individual slices, because linear skull fractures oriented precisely in the plane of CT section and concurrent trauma to the upper cervical spine may occasionally be imaged only on the scout view (Figs. 25-7 and 25-8). Linear fractures do not require specific treatment. However, they do indicate that substantial force was delivered to the skull, so detection of such fractures mandates careful search for accompanying intracranial contusion and hemorrhage. Linear fractures that cross the vascular grooves for the middle meningeal artery or dural venous sinuses are especially likely to cause epidural hematomas.
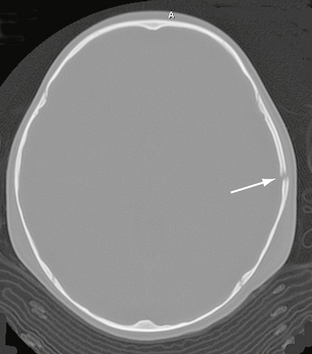
FIGURE 25-5 Linear skull fracture. A radiolucent line (arrow) is seen in the squamous temporal bone on bone window CT.
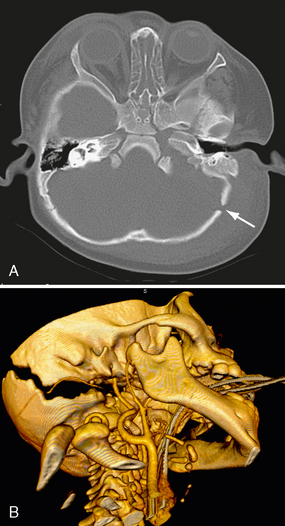
FIGURE 25-6 Diastatic lambdoid fracture in a 1-year-old boy. A, On bone window axial CT, the lambdoid suture is widened (arrow). B, 3D reformatted CT shows well-demonstrated fracture.
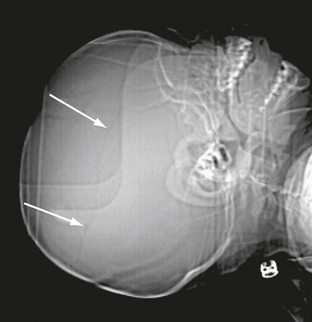
FIGURE 25-7 Linear skull fracture seen on CT scout image only (arrows). Fracture was in the CT scan plane.
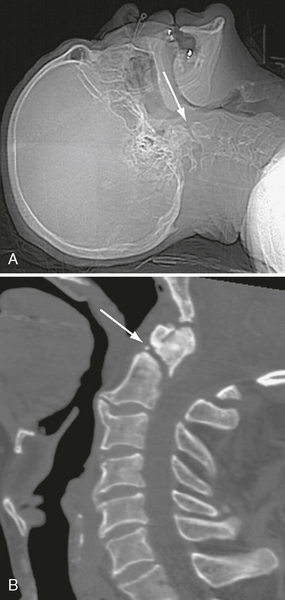
FIGURE 25-8 C2 fracture that was missed on CT head scan. A, Scout radiograph shows fracture line (arrow). B, Follow-up CT of cervical spine demonstrates fracture (arrow).
Depressed fractures are readily seen on CT and are likely to be associated with underlying brain injury. Depressed fractures assume two forms: (1) the adjacent fragments may be angled inward at the fracture line without losing their relative position (Fig. 25-9) or (2) a section of bone may be displaced deep to the neighboring bone (subducted) leaving an open donor site and a double-thickness subduction zone (Fig. 25-10). Fractures depressed more than one full thickness of the skull need to be elevated,24 so the degree of depression should be indicated in the report. Depressed fractures are most common in the frontal and parietal regions. Depressed fractures at the midline, crossing the superior sagittal sinus, carry risk for sinus thrombosis and subsequent venous infarction or hemorrhage. Infection, cerebrospinal fluid (CSF) leak, and post-traumatic epilepsy are other complications of depressed fractures.25
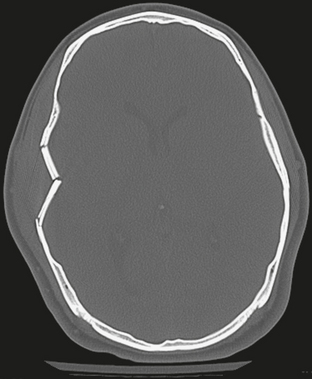
FIGURE 25-9 Depressed right squamous temporal bone fracture. Fragments are bent inward but retain their relative position to the skull.
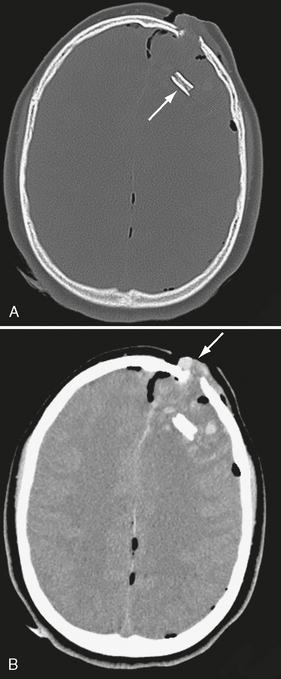
FIGURE 25-10 A 13-year-old male following an ATV accident. A, Note subducted bone fragment (arrow) embedded deeply in frontal lobe. B, Soft tissue windows show brain tissue (arrow) herniating out of the bony defect. Pneumocephalus is present. ATV, All terrain vehicle.
Basal skull fractures often require multiplanar thin-section CT for detection and display of their full extent. Axial slice thicknesses of 1 to 2 mm and coronal reformatted images are recommended. Cranial nerve injury and CSF rhinorrhea/otorrhea are common complications (Fig. 25-11). Pneumocephalus may follow fractures involving the frontal and sphenoidal sinuses (Fig. 25-12) or the mastoid portions of the temporal bones. Air-fluid levels in the paranasal sinuses suggest possible skull base fracture. Longitudinal fractures along the temporal bone may cause ossicular chain disruption (Fig. 25-13). Transverse fractures across the temporal bone may damage the seventh or eighth cranial nerves (Fig. 25-14).26 Fractures of the cribriform plate may cause olfactory nerve injury and anosmia. The optic nerves, cavernous sinuses, and carotid arteries are susceptible to injury from sphenoid fracture. In cases of fracture through the carotid canal, CT angiography or MR angiography should be considered to rule out carotid dissection. Epidural abscess is a potential complication of fracture of the posterior wall of the frontal sinus or mastoid air cells. Meningitis, subdural empyema, and intracranial hypotension can result from meningeal tears.
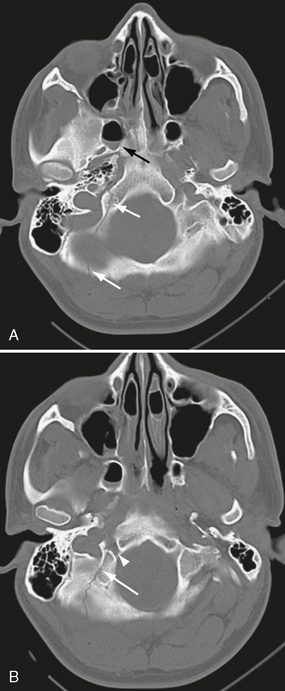
FIGURE 25-11 Posterior skull base fracture in a 21-year-old man. A, Fracture line extends through occipital bone on right (white arrows) and anteriorly into right sphenoidal sinus (black arrow), which has an air-fluid level. B, Fracture involves the right hypoglossal canal (arrowhead).
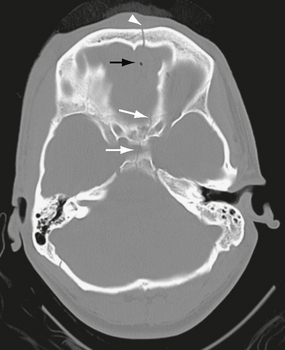
FIGURE 25-12 Anterior skull base fracture. White arrows indicate fracture through the sella turcica and planum sphenoidale. A fracture through the frontal bone is also seen (arrowhead). There is a small amount of pneumocephalus (black arrow).
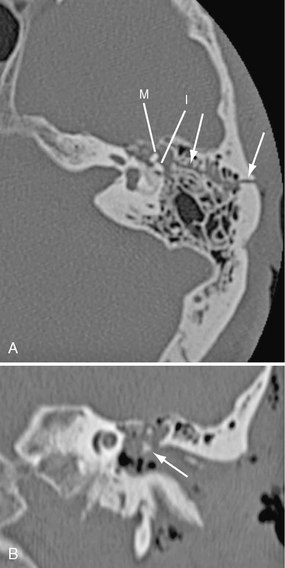
FIGURE 25-13 Longitudinal left temporal bone fracture in a 56-year-old man. A, Axial CT shows fracture line (arrows) extending from mastoid into middle ear cavity. Incus (I) is dislocated from malleus (M). B, Coronal reformatted CT shows dislocated incus (arrow) in mesotympanum.
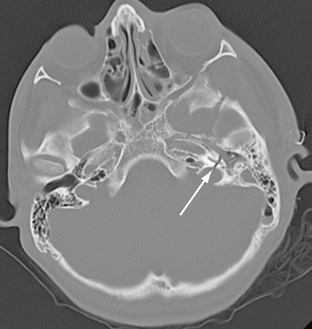
FIGURE 25-14 Transverse left temporal bone fracture in a 15-year-old boy. Axial CT demonstrates fracture through basal turn of the cochlea (arrow), resulting in sensorineural hearing loss.
Growing Skull Fracture
Also known as a leptomeningeal cyst, this lesion occurs exclusively in childhood; 90% are in children younger than age 3 years.27–29 Growing fractures result when the dura deep to the fracture is torn and retracts, exposing the bony margins of the fracture to the pulsations of the arachnoid and subarachnoid CSF. Continued pulsations then erode the bone margins, enlarge the fracture, and permit the arachnoid and subarachnoid CSF to protrude into and through the enlarging fracture line. The presence of meninges in the fracture line interferes with osteoblastic activity and prevents fracture healing.30 Growing skull fractures often originate from a diastatic parietal fracture but may develop at any site with fracture and torn dura. On plain radiographs, leptomeningeal cysts appear as elongate to ovoid radiolucent areas with smooth margins (Fig. 25-15). CT confirms the presence of a CSF-density cystic area at the fracture site (Fig. 25-16). Underlying brain injury is common, as are neurologic deficits, such as a contralateral hemiparesis.
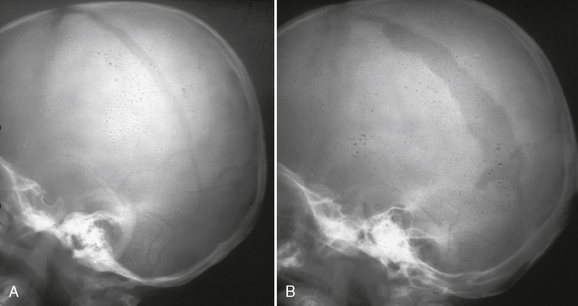
FIGURE 25-15 Growing skull fracture. A, Diastatic fracture of left parietal bone. B, Skull radiograph 31/2 months later shows widening of the fracture line.
(Courtesy of Sandra Fernbach, MD.)
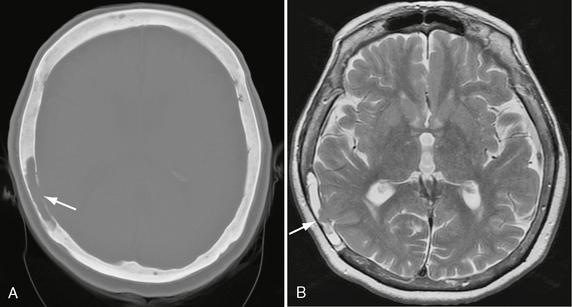
FIGURE 25-16 Intradiploic leptomeningeal cyst in a 73-year-old woman. A, Axial bone window CT shows radiolucent defect in inner table of right parietal bone, with cavity indicated as CSF density in diploic space (arrow). B, Axial T2W MR image shows temporal lobe cortex (arrow) herniating into diploic CSF-filled space.
Leptomeningeal cyst may be a sequela of a form of pediatric closed-head trauma known as the cranial burst fracture. This severe head injury consists of a widely diastatic fracture associated with dural laceration and extrusion of brain tissue outside the calvaria beneath an unbroken scalp.31 The herniated cerebral tissue and dural tear may not be apparent on CT and require MRI for diagnosis.28 Surgical repair of the defect is recommended to prevent further neurologic deterioration.29 Because of its therapeutic implications, some investigators recommend MRI for all skull fractures with more than 4 mm of diastasis to exclude brain hernia. If the dura is intact, the fracture will heal and a leptomeningeal cyst will not develop.27
EXTRA-AXIAL HEMORRHAGE
Epidural Hematoma
Epidemiology
Hemorrhage into the potential space between the periosteal dura and the inner table of the skull is seen in 2.7% to 4% of all traumatic brain injuries.32 The leading causes of EDH are motor vehicle accidents, falls, and assaults, in that order. Associated skull fractures are present in 75% to 90% of cases. EDHs arise from tearing of a middle meningeal artery, middle meningeal vein, diploic vein, or dural venous sinus. Most EDHs occur in the squamous temporal region (Table 25-4), where the skull is thin and easily fractured, tearing the middle meningeal artery (Fig. 25-17). Frontal and parietal EDHs are less common. Because the dura is tightly adherent to the skull, epidural collections tend to be short and elliptical (Fig. 25-18). They seldom cross suture lines, where the dura is firmly attached to the inner table, unless the fracture also traverses the suture. Up to 50% of EDHs are accompanied by other intracranial injury, most commonly subdural hematoma, cortical contusions, and diffuse brain swelling. Less than 5% of adult EDHs occur in the posterior fossa.
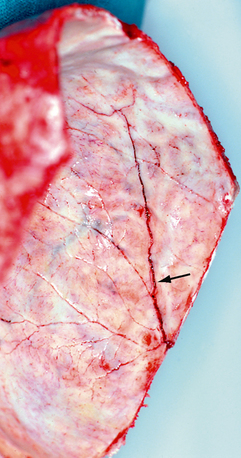
FIGURE 25-17 Postmortem photograph shows a linear fracture line (arrow) crossing the grooves for the ascending branches of the middle meningeal artery (internal view of Figure 25-4A).
(Courtesy of John Deck, MD.)
Clinical Presentation
About half of all EDH patients suffer a period of loss of consciousness, followed by a lucid interval and then subsequent neurologic deterioration as the hematoma expands. If there are other intracranial injuries, the victim may remain unconscious from the time of the accident. In one series of 200 surgically treated EDHs, 23% had no loss of consciousness preoperatively.33 Those individuals who remained conscious up to the time of surgery had a favorable outcome (only 4% unfavorable outcome) compared with those who were unconscious up to the time of surgery (10% mortality, 23% unfavorable functional recovery). Functional outcome was highly correlated with the GCS score at the time of admission (see Table 25-1). Other presenting symptoms include focal neurologic deficit, decerebrate posturing, and seizures.32
Imaging
CT
On noncontrast CT scans, acute EDHs typically appear as biconvex, hyperdense masses adjacent to the inner table of the skull (Figs. 25-19 and 25-20). The CT attenuation of blood is related to the high electron density of the hemoglobin molecule and not the iron content. Clotted blood has a hematocrit of about 90%, whereas unclotted blood has a hematocrit of about 50%. On CT, areas of decreased attenuation within an EDH are thought to represent unclotted blood and indicate active bleeding (the swirl sign) (Fig. 25-21).34 EDHs that result from a torn venous sinus may cross the falx or tentorium (Fig. 25-22) and thus be present in both the infratentorial and supratentorial compartments.35
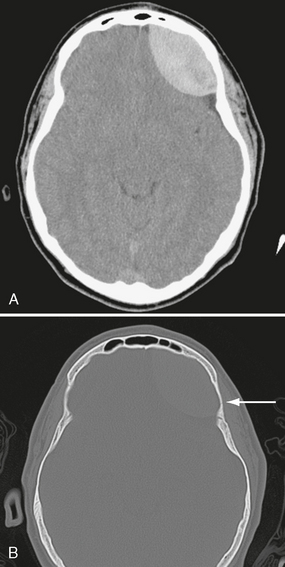
FIGURE 25-19 Epidural hematoma in an 18-year-old boy. A, Axial CT shows high-density biconvex collection in left frontal region. B, Bone window CT shows subtle linear fracture (arrow).
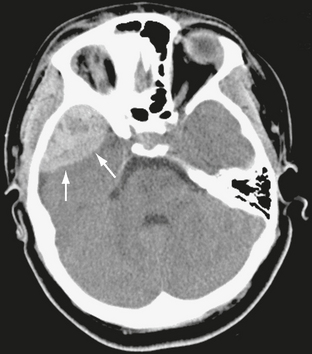
FIGURE 25-20 Epidural hematoma in a 16-year-old girl. Axial CT demonstrates high-density collection in the right middle cranial fossa (arrows).
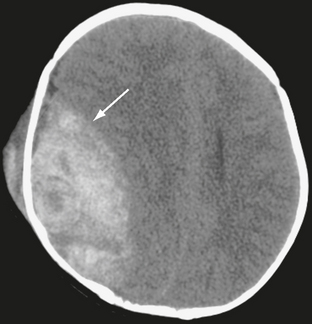
FIGURE 25-21 Epidural hematoma in a 5-year-old boy. Unenhanced CT. Arrow indicates interface between brain and hematoma. Lower-density areas within the hematoma are the result of active bleeding (the swirl sign).
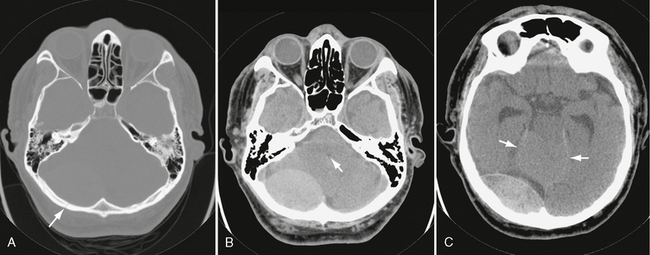
FIGURE 25-22 Venous epidural hematoma from transverse sinus laceration. A, Bone window CT shows linear fracture of occipital bone (arrow). B, CT through posterior fossa demonstrates large infratentorial hematoma on right. Fourth ventricle (arrow) is compressed and displaced. C, Higher slice shows supratentorial component of hematoma. Upward herniation of cerebellar vermis (arrows) through the tentorial incisura is seen.
MRI
On MRI, acute EDHs are usually isointense to brain on T1-weighted (T1W) images and isointense to hypointense on T2-weighted (T2W) images. Early subacute EDHs are hyperintense on T1W images and hypointense on T2W images (Fig. 25-23). Late subacute EDHs are typically hyperintense on both T1W and T2W imaging. The medially displaced dura is seen as a black line between the hematoma and compressed brain. MRI is rarely necessary to make the diagnosis of EDH, except occasionally for those that are located at the midline vertex, which are difficult to appreciate on CT.
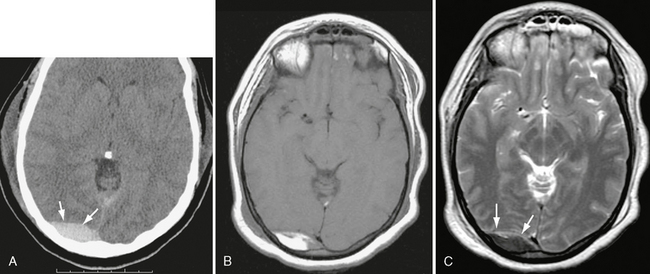
FIGURE 25-23 Venous epidural hematoma, acute to early subacute phase. A, Unenhanced CT, day 2 post injury. High-density collection is seen in right occipital region (arrows). B, T1W MRI, day 4. Note bright signal in hematoma. C, T2W image shows low intensity in hematoma (arrows), consistent with intracellular methemoglobin.
Management
The management of EDHs has changed dramatically in recent decades.36 Before CT almost all EDHs were surgically evacuated. Now they can be followed with serial imaging studies, so more than 60% of EDHs can be managed nonsurgically.37 Published neurosurgical guidelines specify which patients are suitable for nonoperative management32:
• An EDH greater than 30 mL should be surgically evacuated regardless of the patient’s GCS score.
• An EDH less than 30 mL and with a thickness less than 1.5 cm and with a midline shift less than 5 mm in patients with a GCS score greater than 8 without focal deficit can be managed nonoperatively with serial CT and close neurologic observation in a neurosurgical center (Fig. 25-24).32
• The volume of an EDH can be approximated from the CT scan, using the formula V = 0.5 × L × W × H.
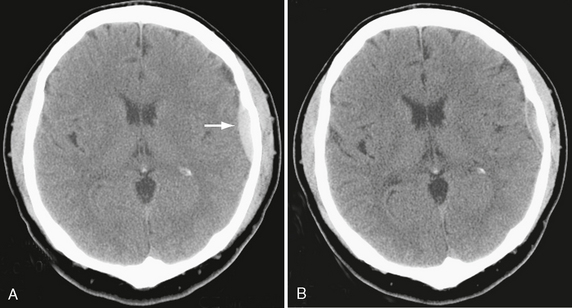
FIGURE 25-24 Nonoperative management of a small epidural hematoma in a 29-year-old man. A, Initial CT demonstrates acute left frontal convexity hematoma (arrow). B, Follow-up CT shows resolving hematoma.
A lower threshold is used for operative management of middle cranial fossa EDHs, because these are more likely to cause uncal herniation and brain stem compression. Posterior fossa EDHs are usually evacuated, because mass effect in this compartment is poorly tolerated.38
In a study of 160 cases of EDHs treated conservatively, Sullivan and coworkers found that 23% enlarged, with a mean increase of 7 mm in width. The mean time to enlargement was 8 hours after injury. No EDH enlarged after 36 hours.39 Of the EDHs that enlarged, 43% required surgical evacuation. Hence, close monitoring with CT is of paramount importance in the first 36 hours and especially in the first 8 hours. EDHs begin to decrease in size after the second week and in most patients will disappear completely after 4 to 6 weeks.
Subdural Hematoma
Subdural hematomas (SDHs) are misnamed because they actually represent hemorrhage into the innermost layer of the dura, designated the dural border cell layer. They are not hemorrhages into a potential subdural space, because there is no potential subdural space.40,41
Epidemiology
Subdural hemorrhages are seen in 12% to 29% of individuals with severe traumatic brain injury.42 As with EDHs, the most common mechanisms of injury are motor vehicle accidents, falls, and assaults. In patients older than age 75 years, a fall is the most common cause of SDH.
Pathophysiology
SDHs arise from three sources: (1) rupture of a bridging cortical vein, (2) hemorrhagic contusion that breaks through the arachnoid, and (3) tear of a cortical artery or vein.43 Most acute SDHs that are not associated with other intracranial injuries are thought to result from bridging vein rupture. Angular acceleration/deceleration of the head in the sagittal plane is the most common causative factor.44 The bridging cortical veins course anteriorly and at an angle from the cortical surface of the frontal, parietal, and occipital lobes to the superior sagittal sinus (Fig. 25-25). This makes them particularly prone to rupture from a fall onto the occiput. The portion of the vein in the dural border cell layer is thinner and more fragile than the subarachnoid segment, which accounts for the more frequent hemorrhage into the subdural compartment.43 The dural border cell layer is composed of cells oriented like stacked plates with few cell-to-cell connections. For that reason it shears easily between cells along the plane of the layer. In the swine model, intracranial subdural hemorrhages may shear far caudally into the spinal canal in minutes.45 It appears to be easier to shear along the dural border cell layer than to separate the periosteal dura from the inner table, so subdural hemorrhages typically appear to extend far more widely than do EDHs and typically spread over the surfaces of the hemispheres, along the falx, and under the occipital and temporal lobes (Fig. 25-26
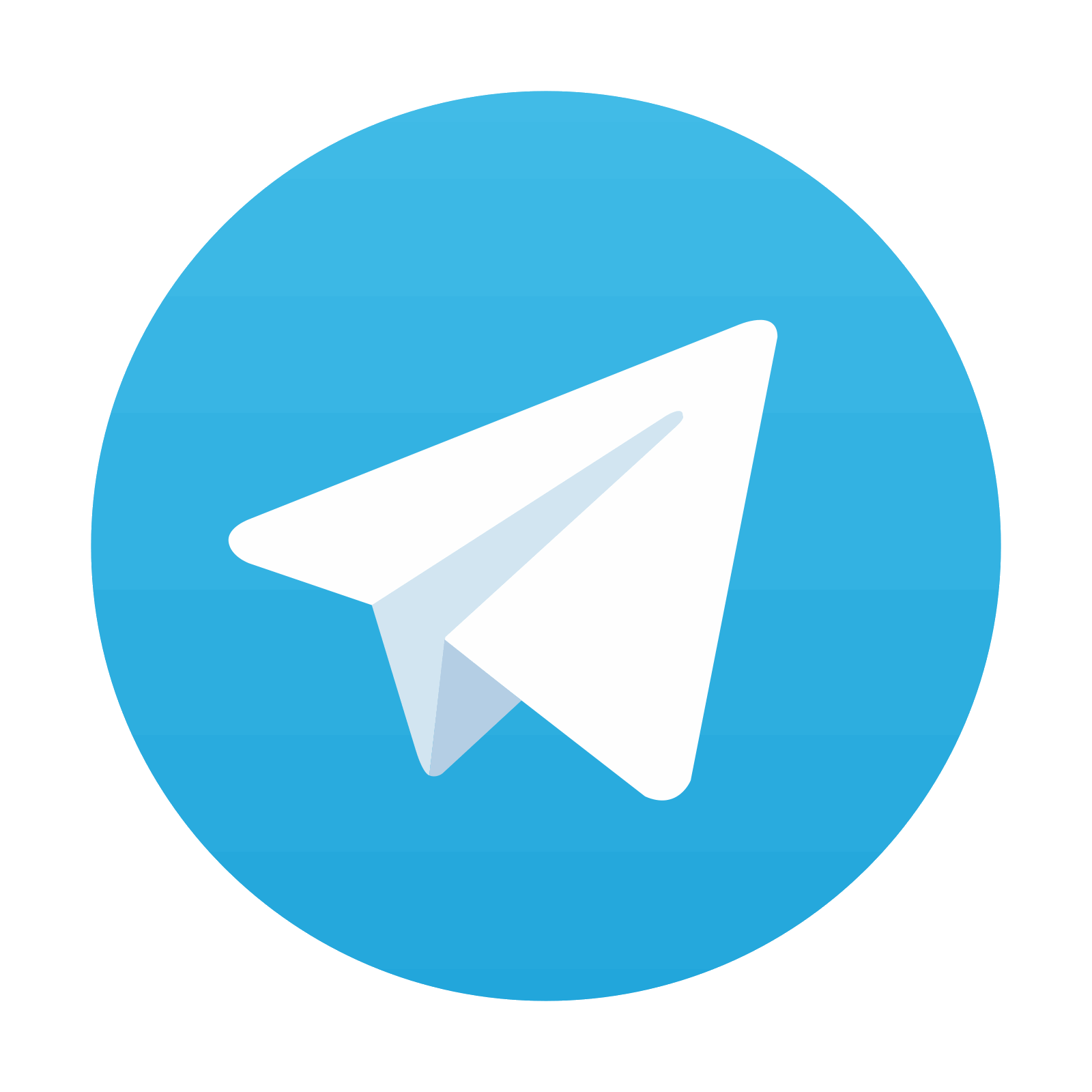
Stay updated, free articles. Join our Telegram channel
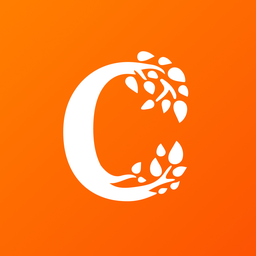
Full access? Get Clinical Tree
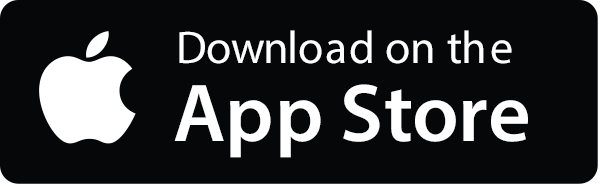
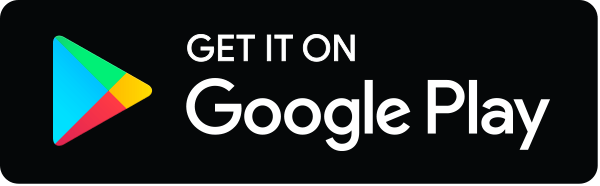