Fig. 45.1
(a) Schematic drawing of patient’s head fixated with a stereotactic frame (yellow) and positioned inside the half spherical ultrasound transducer (gray). Cool degassed water (blue) is circulating around the head. The entire focused ultrasound device is inserted into the bore of a 3 T MR scanner (light gray). (b) Patient positioning as described in (a)
Pretreatment Planning
The intervention planning is of great importance, involving magnetic resonance neuroradiological examination of the anatomy at the target location and of adjacent brain structures. Days before treatment, patients receive a high-resolution CT scan (512 × 512 matrix, 1 mm slice thickness) covering the entire cranium. The CT serves for screening for intracranial calcifications that have to be excluded from the beam path and for co-registration with the planning 3D-T1 MRI (see below). Additional MRI examinations are done for intervention planning, especially for determining the procedural approach, for studying the brain anatomy, and for checking for possible pathologies that would exclude the patient from the study.
On the day of the treatment, patients are given a benzodiazepine as a slight sedative and esomeprazolum for stress ulcer prophylaxis. Treatment begins with positioning the patient in the ExAblate 4000 system integrated to the patient table of the 3 T MR scanner and filling the gap between the head and the transducer with degassed water which circulates at a temperature of 16 °C. Calibration of the coordinate systems of the FUS device and the MR scanner is followed by a series of stereotactic MR images obtained while the patient is in the proposed treatment position. First, the anterior and posterior commissures are delineated on midsagittal T2-weighted MR images (T2WI). This allows a stack of axial T2WI and 3D-T1WI to be positioned parallel to the ac-pc plane, as well as orthogonal coronal T2WI. The MR images are then co-registered to the previously obtained CT and MRI scans, which are uploaded onto the treatment console, and aligned according to the transducer coordinates for system calibration. The co-registered CT scan also serves for adjustment of phase and amplitude of the ultrasound beams for each of the 1,024 transducer elements, using the acoustic model of the ExAblate 4000 software (see above). The coordinates of the target, i.e., the posterior part of the central lateral nucleus (CLp) of the thalamus, are localized days before the intervention on 3D-T1WI using the multiarchitectonic Morel atlas of the human thalamus and basal ganglia [34]. These coordinates are used to mechanically place the focal point of the ultrasound transducer in such a way that it roughly matches with the target position. On the treatment day, the target coordinates are entered into the planning software in order to calibrate the FUS system and for fine image-guided adjustment on the co-registered MR images that are obtained while the patient is in the proposed treatment position inside the transducer. In addition, these MR images are used for intraprocedural monitoring and for postprocedural treatment validation.
The responsible physicians review the high-resolution MR images on the system workstation; check the provisional beam path for possible obstacles, such as air-filled sinuses or tissue calcifications; and select appropriate MR images for treatment planning and for visualization of the exact anatomy during the intervention.
Intervention Procedure
After the therapy planning software has calculated the parameters required for thermally ablating the defined target region, the FUS intervention procedure starts. The ultrasound transducer transiently generates a point of focused ultrasound energy, called a sonication, according to the system parameters set by the operator. First, several subthreshold, non-coagulative low-energy sonication steps are initiated, to identify the focus on thermal images and to verify the target location. In order to accomplish this, the applied energy and the sonication duration are chosen in a way that the focal temperature at the target location increases only minimally. Repeated sonications, each lasting typically between 15 and 25 s, elevate the tissue temperature at the focus within a well-defined region of a few millimeters in diameter in a stepwise fashion to a level of 39–42 °C, which is well below the temperature needed for protein denaturation and tissue coagulation [35]. The focal temperature rise can be monitored on quantitative temperature maps that are based on the proton-resonance frequency shift. A series of thermomaps is displayed in real time and updated at a rate of 3–4 s. These maps can be used as surrogates for viable tissue and allow precise target verification without damaging the tissue by determining the position and size of the hot spot, allowing for electronically steered fine geometric corrections of the focus if necessary, and assessing the overall safety profile of the applied sonication parameters. In addition, the thermal images are screened for secondary hot spots especially in the neighborhood of the targeted focus and at the skull and brain surface.
This exact and noninvasive identification of the thermal spot at the target localization, therefore, is the key to the safety and accuracy of the focused ultrasound intervention procedure (Fig. 45.2).
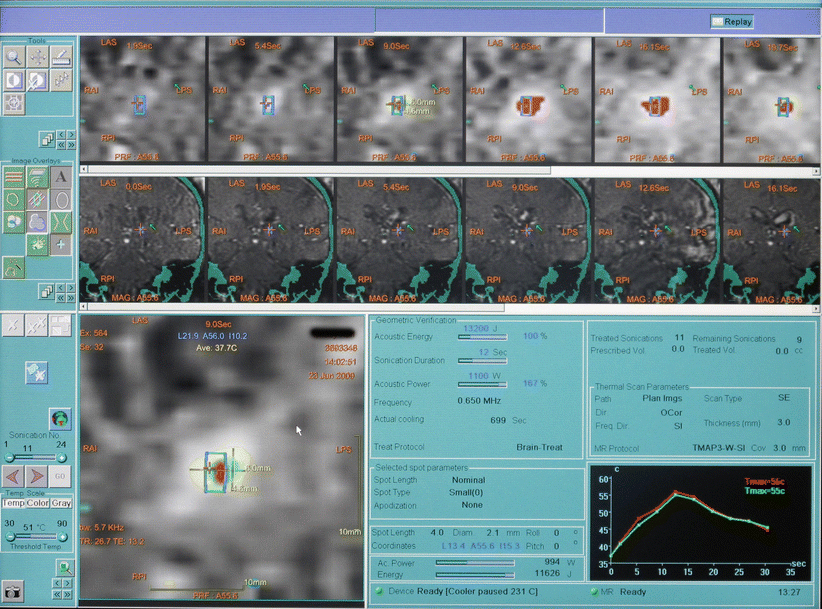
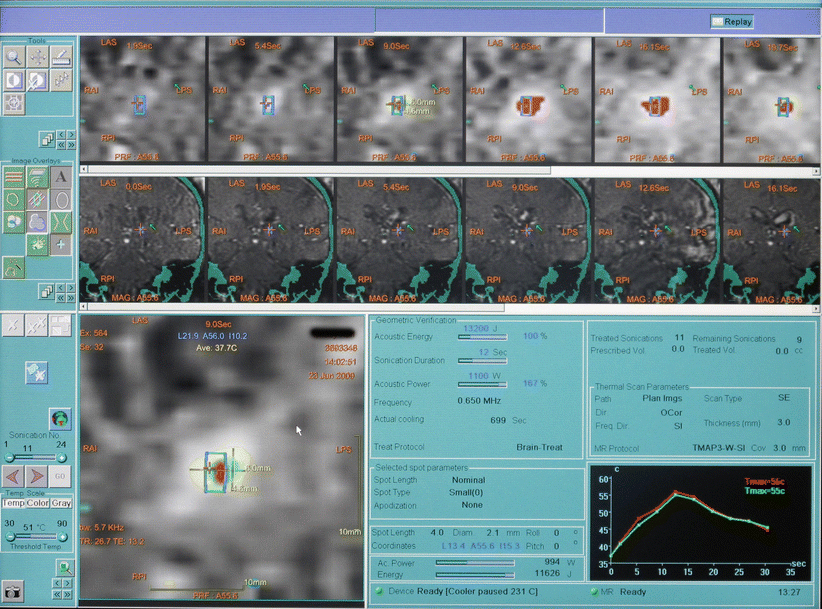
Fig. 45.2
Screenshot of FUS console. Top row: thermal maps taken every 3.5 s. Second row: magnitude images. Bottom left: hot spot with thermal contours. Bottom right: temperature evolution at the target
Since patients are awake during the entire FUS intervention, the cooling intervals of several minutes duration between each sonication can be used to question the patient and examine him or her neurologically. This precoagulation phase is well suited to test the site to be treated functionally by blocking or stimulating nerve conduction comparable to transcranial magnetic stimulation, but with great advantage of precise localization [36]. During sonications at medium temperatures around 45 °C, patients are typically reporting vestibular symptoms, such as vertigo, flying high, falling back, or slight nausea, when the CLp is exactly targeted. While such neuromodulation serves as an additional important information for exact targeting, it also allows for monitoring unwanted somatosensory excitations, such as newly appearing pain, dysesthesia or paresthesia, or even motor symptoms that would arise from touching the sensory VPM/VPL or the motor VL nuclei, respectively. Such signs would alert the physician to readjust the target location before performing the final ablative intervention. Once the target is verified, the operator chooses the acoustic energy by adjusting the power and the duration of sonication, in order to raise the temperature at the focus up to 58–62 °C, resulting in a spot of thermally coagulated tissue of typically 3 × 3 mm in diameter and 4 mm along the beam path (Fig. 45.2).
In order to induce precise focal tissue ablations in a safe manner, about six to nine continuous wave sonications of 15–25 s duration with a stepwise increased acoustic power of 1,200 W resulting in 15,000–18,000 J of total energy are applied. The thermal effects of a sonication on brain tissue are dependent on the induced temperature evolution [37]. Cell death, however, might occur at significantly lower temperature, depending on the length of ultrasound exposure. Therefore, the ExAblate system computes the accumulated thermal dose at each target location online based on the information from the achieved temperature profile and the duration of the sonication using the Sapareto-Dewey equation [38, 39]. In animal experiments, it has been documented that the thermal dose is a significant predictor or tissue damage. The magnitude, size, and distribution of the thermal dose are critical, since they not only ensure therapeutic efficacy, such as thermal coagulation necrosis, but they also inform the operator immediately where tissue damage occurs and where not. From in vivo experiments on animal brains, it could be demonstrated that a thermal dose threshold of 17 cumulative equivalent minutes (CEM) at 43 °C results in cell coagulation with a probability of 50 % [40]. Therefore, unlike other imaging modalities, MR thermometry permits real-time intraprocedural closed-loop feedback of thermal ablation, thus guiding the treatment procedure and determining the treatment end points.
An emergency button is attached to the patient’s hand, allowing him to stop the ongoing process at any time during the intervention. In addition, pulse, blood pressure, and oxygen saturation are continuously monitored in all patients.
The absence of any trajectory restriction by the FUS method compared to any other method that uses invasive instrumentation is of the utmost advantage to the operating physician as well as to the patient. Therefore, depending on the reaction of the patient in terms of immediate pain relief following the first ablative sonication, the procedure can be repeated using the identical parameter settings at multiple (usually one to three) adjacent target points or to the homotopic location at the contralateral side of the thalamus.
Postoperative Evaluation and Follow-Up
Following the last sonication, the patient is taken out of the scanner and the fixation frame is removed. If tolerated, a series of MR images, e.g., plain and contrast-enhanced T1WI as well as T2WI, is obtained in the standard head coil immediately after the intervention. On postcontrast MRI, a ring enhancement of 1–2 mm bordering the lesion can be seen, indicating that the blood–brain barrier is disrupted. This enhancement disappears almost completely within 48-h postintervention. The non-enhancing central part of the lesion signifies tissue necrosis and blocked capillaries induced by thermal ablation [41] (Fig. 45.3).
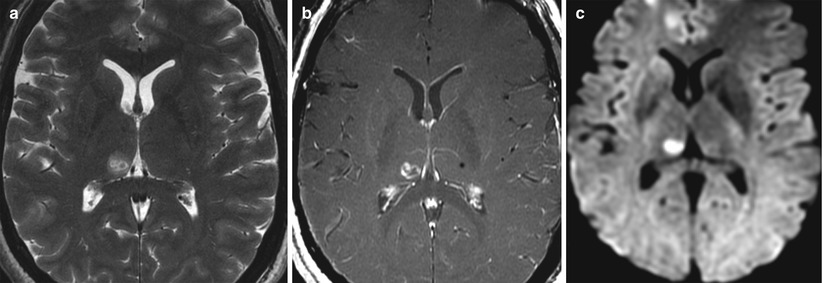
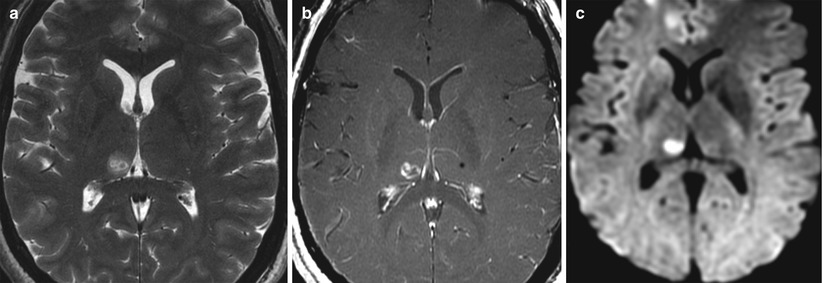
Fig. 45.3
Axial MRI of the patient’s brain taken immediately after a FUS intervention in the right medial thalamus (two therapeutic sonications in right CLp). (a) Hyperintense lesion on T2-weighted MRI with hypointense center and faint hyperintense perifocal edema of a few millimeters. (b) T1-weighted postcontrast MRI with ring enhancement. (c) Cytotoxic edema on DTI
The extent of the sonication lesions is best seen at 48-h postintervention, after which they start to shrink. They are well demarcated and hypointense on T1WI and somewhat heterogeneous on T2WI, appearing strongly hyperintense with a hypointense center, surrounded by a perilesional and rather fuzzy hyperintense concentric zone indicating a perifocal vasogenic edema of only a few millimeters in size (Fig. 45.4).
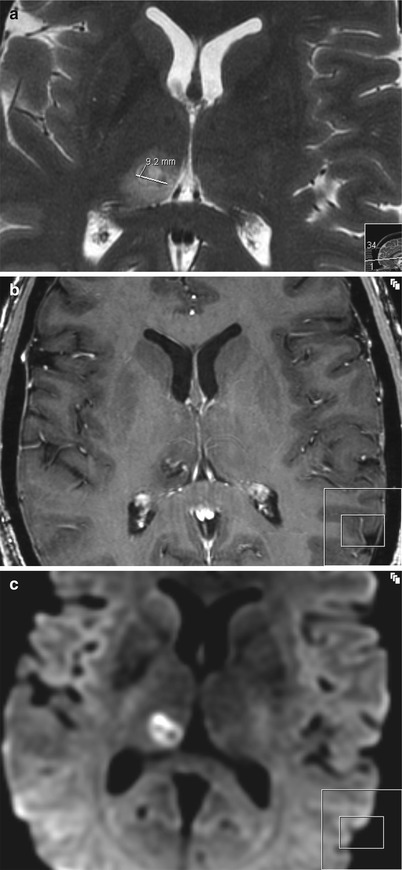
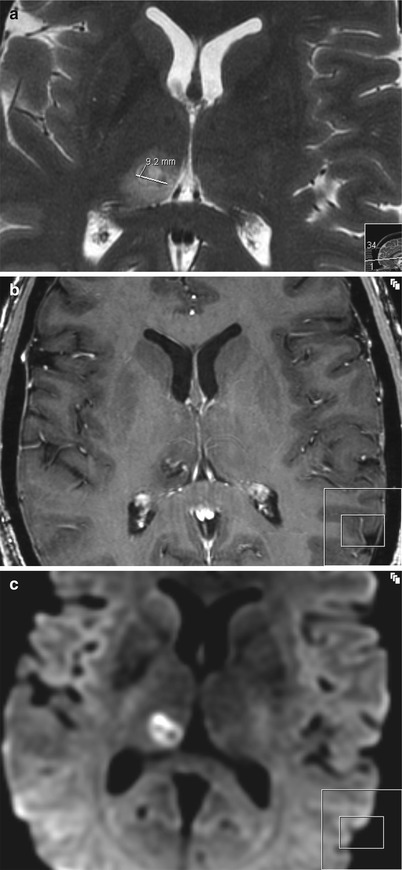
Fig. 45.4
Axial MRI (same sequences as in Fig. 45.3) taken 48 h after FUS intervention. (a) At 48 h, the vasogenic edema has its largest extension on T2WI. (b) No or minimal contrast ring enhancement indicating that the blood–brain barrier is closed at 48 h. (c) Ring-like cytotoxic edema still visible
The lesions are disclike on axial images of 3–4 mm diameter and elliptic in coronal and sagittal slices, measuring 4–5 mm in length. The cytotoxic edema covering the entire lesion stands out as blooming hyperintensity on isotropic diffusion-weighted images. This DTI hyperintensity lasts for several days, but is not visible at 1 month follow-up, where the lesion appears hypointense comparable to the T2WI.
Since high-intensity focused ultrasound produces heat-induced coagulation necroses, the lesions are immediately set, with only a slight transient cytotoxic edema and minimal tissue swelling. No major tissue displacement occurs.
By comparing the planned target coordinates with coordinates of the center of the sonication lesion at 48-h postintervention, the precision can be estimated with great accuracy. The preliminary experience with treated patients to date confirms what is expected from animal experiments that the FUS intervention is highly precise and accurate with an exactness of below 1 mm in all three dimensions.
On follow-up over a period of 3–12 months, the sonication lesions shrink in size by almost 50 %. It is important to mention that the extent of these lesions never expands, i.e., it never “grows” over time, as is usually observed with lesions produced by ionizing radiation (Fig. 45.5).
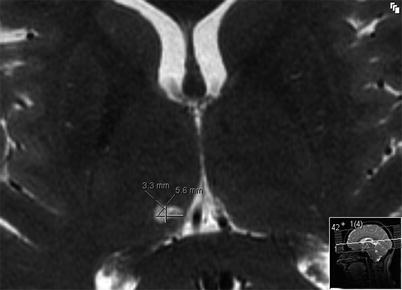
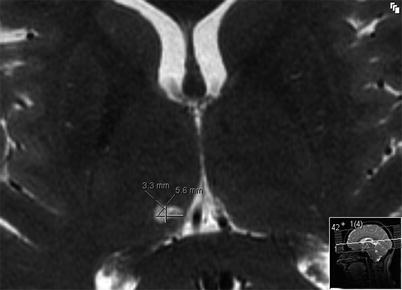
Fig. 45.5
Axial T2-weighted MRI taken at 3-month follow-up show shrinking of the thermal lesions in the right medial thalamus (CLp) over time
The long-term effectiveness and risks of the FUS intervention procedure in patients with functional brain disorders have to be evaluated by thorough neurological testing on a large cohort over an extended period of time. In this regard, the 1-year follow-up evaluation of the first 12 treated patients with chronic neuropathic pain looks promising with a mean overall pain relief of 60 %. As expected from experiences with radio-frequency ablation in this patient population, there are patients with 100 % therapeutic success and others that barely profit from the intervention and are still suffering substantially. However, in some of these suffering patients, close relatives report on improved mood changes and less dependence on pain medication, an observation that often is less appreciated by the patients themselves.
Summary and Future Trends
For many years, treatments of brain tumors and functional brain disorders with focused ultrasound required removal of the skull bone from the sonication pathway [42]. However, this made the procedure invasive, with added risks and increased costs. The assumed necessity of craniectomy has prevented MRgFUS from being widely tested in the brain, despite its benefits in comparison with other noninvasive techniques such as stereotactic radiosurgery.
The transcranial (tc) approach of magnetic resonance-guided focused ultrasound surgery (MRgFUS) is, therefore, a long expected completion of a noninvasive neurosurgical intervention procedure. As such, tcMRgFUS is an emerging alternative neurosurgical treatment modality, especially for functional brain disorders, with real-time temperature monitoring and visual control of the entire intervention process. Results from numerous animal experiments and ongoing clinical trials promise clear and unambiguous advantages over other treatment modalities, such as open cranial surgery, radiosurgery, deep brain stimulation, and other thermo-ablative procedures. Because FUS interventions are highly precise with a steep thermal gradient to non-focal tissue, damage to collateral brain tissue is almost nonexistent, while the risk of infections or hemorrhage related to the procedure is very low. Absence of trajectory restrictions, instrumentation, and ionizing radiation allows repeated FUS applications to eradicate pathological tissue completely in a single session or in subsequent sessions. There is no danger of late secondary tumor formation as a consequence of FUS treatment, as it is in radiosurgery or radiotherapy.
Is there a revival of old ablative procedures for treating functional brain disorders? This question cannot yet be answered with confidence. However, modern MR imaging modalities with detailed anatomical delineation, the availability of an exact multiarchitectonic stereotactic atlas [34], MR image guidance of the whole FUS intervention procedure, the possibility of online temperature monitoring, and last but not least the remarkably improved neuroanatomical and neurophysiological knowledge of the thalamocortical interaction and its pathology in functional brain disorders make transcranial FUS as a new, precise, and noninvasive neurosurgical intervention modality very attractive. In addition, the FUS intervention can be repeated as often as necessary, and the treated patients can be examined with MR imaging during future diagnostic work-up, since no instrumentation and no metal are implanted into the brain.
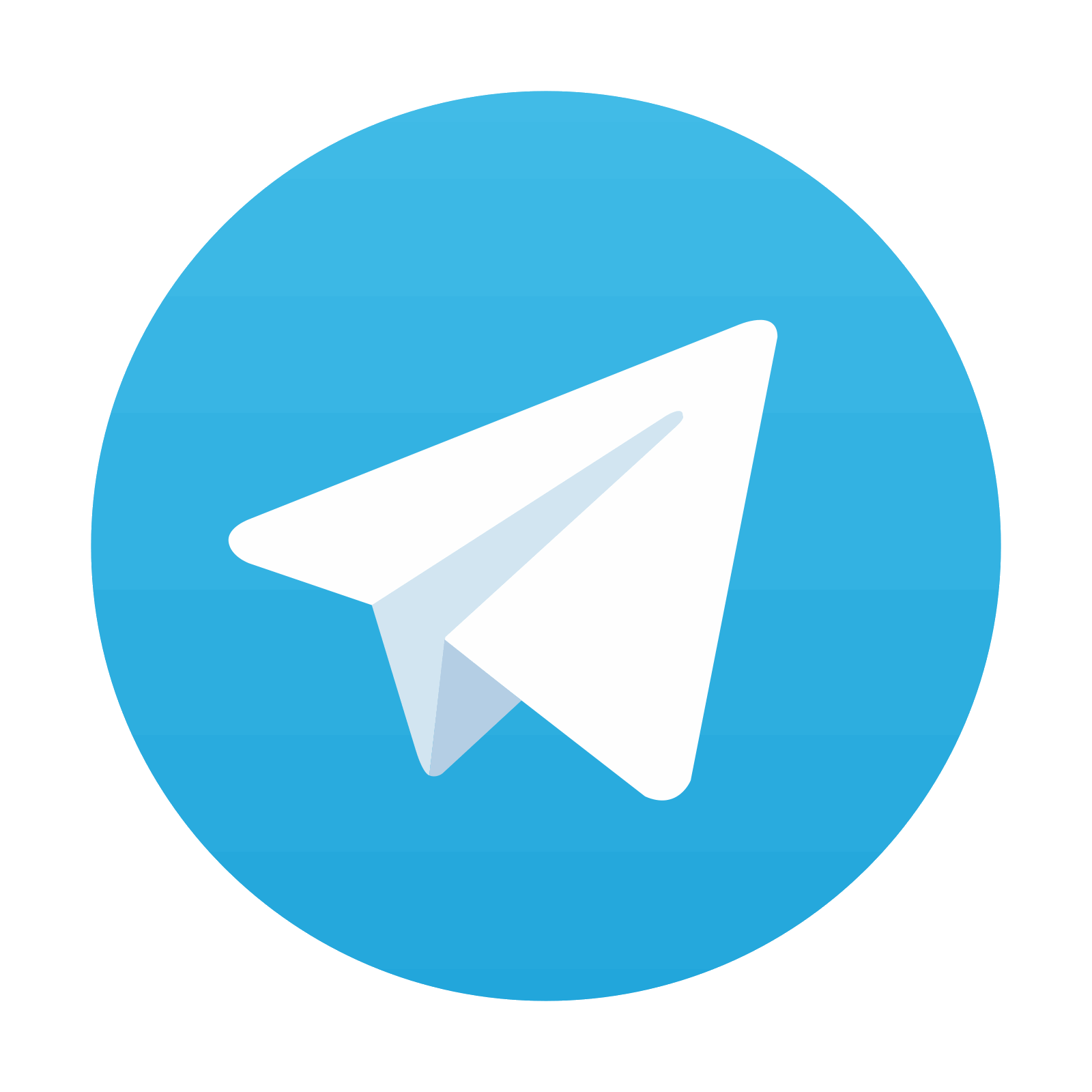
Stay updated, free articles. Join our Telegram channel
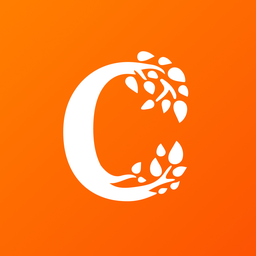
Full access? Get Clinical Tree
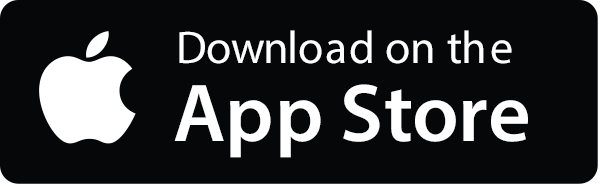
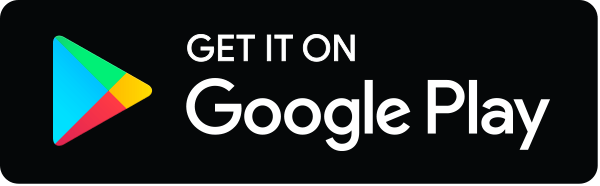