and is fixed as E 1, that is
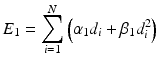
(19.1)
![$$ {E}_0={\displaystyle \sum_{i=1}^N\left[{\alpha}_0\left(\delta {d}_i\right)+{\beta}_0{\left(\delta {d}_i\right)}^2\right]} $$](/wp-content/uploads/2016/11/A322115_1_En_19_Chapter_Equ2.gif)
(19.2)
![$$ {\displaystyle \sum_{i=1}^N\left[{\alpha}_0\left(\delta {d}_i\right)+{\beta}_0{\left(\delta {d}_i\right)}^2\right]\to \mathrm{M}\mathrm{i}\mathrm{n}} $$](/wp-content/uploads/2016/11/A322115_1_En_19_Chapter_Equa.gif)
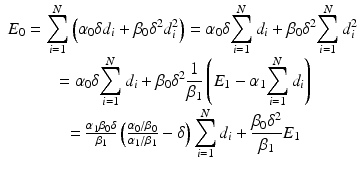
Ultimately, the adjudication can be described as follows:
(i)
if
, hypofractionated irradiation is better than multi-fractionated irradiation.

(ii)
if
, multi-fractionated irradiation with a constant dose is better.

The result does not depend on the value E 1, nor the parameters, α 0, β 0, α 1, β 1, but the ratio
and δ.

The clinical feasibility of this model can be examined assuming two lung tumors with the same volume of 2.0 cm3 but situated in different locations: for example peripheral lung vs. central lung. The organs at risk are normal lung tissue, spinal cord, brachial plexus, pulmonary artery, heart, esophagus, and the proximal bronchial tree [33, 34]. Using SBRT technology, one can assume that the major complication probabilities are related to the central mediastinal structures such as pulmonary artery, heart, esophagus, and the proximal bronchial. α 0/β 0 for the central mediastinal structures is very likely to be smaller than α 1/β 1 for the tumor so that we can assume
is smaller than 1.0. In the treatment of peripheral lung tumors, the central mediastinal structures do not receive any significant dose (δ =0.0). However, in the treatment of central lung tumors, the central mediastinal structures receive nearly the same dose as the target volume (δ =1.0). Consequently, the model predicts that hypofractionated radiotherapy is preferable for the peripheral tumor and multi-fractionated irradiation is preferable for the central tumor. These preferences are consistent with clinical findings for SBRT of stage I squamous cell carcinoma of the lung. Further refinement of this model must incorporate our understanding of radiobiology as many investigators have demonstrated the importance of parameters such as DNA repair, cell cycle, hypoxia, and reoxygenation.

19.5 Functional Imaging for DCRT
Functional imaging may well be useful in DCRT in order to optimize the dose delivery based upon metabolic activity of the tumor as it relates to the functional capacity of nearby normal tissues. Positron emission tomography (PET) can be used for the determination of these function of normal tissue and abnormal function of cancer cells only if the signal to noise ratio of imaging is sufficient for quantitative analysis [35]. The reproducibility of functional imaging is strongly dependent on the interval between the injection of the probe and the detection of images. Radiation oncologists should work closely with diagnostic radiologist to define a common imaging protocol so that quantitative analysis is comparable between scans. For example, in the detection of tumor hypoxia by functional imaging, accumulation of FMISO following requires 4 h to reach steady state levels. Imaging prior to early after injection of the tracer will result in inaccurate estimation of the degree of tumor hypoxia [36]. When images are acquired 4 h after the injection of FMISO, the detection and reproducibility of the hypoxic areas of tumors are excellent [37]. Since the signal to noise ratio (SNR) of FMISO imaging is much lower than FDG imaging, the efficiency of positron emission tomography (PET) is critical for the quantitative accuracy [38].
19.6 Molecular Technologies Expected to Be Useful for DCRT
Although the incidence of grade III radiation pneumonitis (RP) in SBRT has been quite low after SBRT, the consequences of severe RP are so clinically significant that reliable biologic markers to predict RP before treatment are warranted [26]. Yuan et al. investigated the association between single nucleotide polymorphisms (SNPs) in the transforming growth factor 1 (TGFbeta1) gene and risk of RP. These investigators reported that CT/CC genotypes of TGFbeta1 rs1982073:T869C to be associated with a lower risk of RP compared with the TT genotype [39]. Further study of this SNP as well as the development of other associated SNPS will enhance our predictive models of RP after high dose radiation.
Selection of patients who are most likely to benefit from DCRT alone and those who should receive additional systemic therapy are important clinical decisions that factor into the appropriateness of DCRT. The effectiveness of a local therapy is maximized when the risk of systemic disease is lowest. Measurement of circulating tumor DNA (ctDNA) has been proposed as a method to improve the selection of the patients for DCRT and for systemic therapy [40]. Newman et al. introduced cancer personalized profiling by deep sequencing (CAPP-Seq), an economical and ultrasensitive method for quantifying ctDNA. They implemented CAPP-Seq for NSCLC with a design covering multiple classes of somatic alterations that identified mutations in >95 % of tumors. These investigators detected ctDNA in 100 % of patients with stage II-IV NSCLC and in 50 % of patients with stage I, with 96 % specificity for mutant allele fractions down to approximately 0.02 %. Levels of ctDNA were highly correlated with tumor volume and distinguished between residual disease and treatment-related imaging changes, and measurement of ctDNA levels allowed for earlier response assessment than radiographic approaches. The CAPP-Seq could be routinely applied clinically to detect and monitor diverse malignancies, thus facilitating personalized cancer therapy and improving the therapeutic outcomes.
19.7 Informatics to Use New Radiotherapy Technologies Properly
Scientific, economic, and ethical considerations to prevent inappropriate use of new radiotherapy technology is strongly desired. Process analysis of the physicians’ decision making using advanced technologies in informatics may be useful for this purpose. Widespread adoption of electronic medical records will help to facilitate uniformity and transparency of clinical radiation oncologic decisions. For example, a common clinical scenario is to determine if re-irradiation is feasible in an area of tumor recurrence. Re-irradiation using small treatment volume with stereotactic technology may be clinically feasible for selected patients [41]. However, the long-term biological consequence for re-irradiation of serial organs is largely unknown. DCRT holds great promise to provide exact 4D dose distribution of both the tumor and normal tissues. This knowledge will improve the therapeutic ratio for this technology.
The future of radiotherapy will incorporate advancements in the fields of biology, physics, and imaging. Applying this knowledge to clinical radiotherapy will improve the outcomes for patients. However, rigorous standards must be utilized to select the patients that would be most appropriate for these advanced radiotherapy techniques. Clinicians must work closely with researchers to maximize the benefits of these techniques for our patients.
References
1.
2.
3.
Onishi H, Araki T, Shirato H, et al. Stereotactic hypofractionated high-dose irradiation for stage I nonsmall cell lung carcinoma: clinical outcomes in 245 subjects in a Japanese multiinstitutional study. Cancer. 2004;101:1623–31.PubMedCrossRef
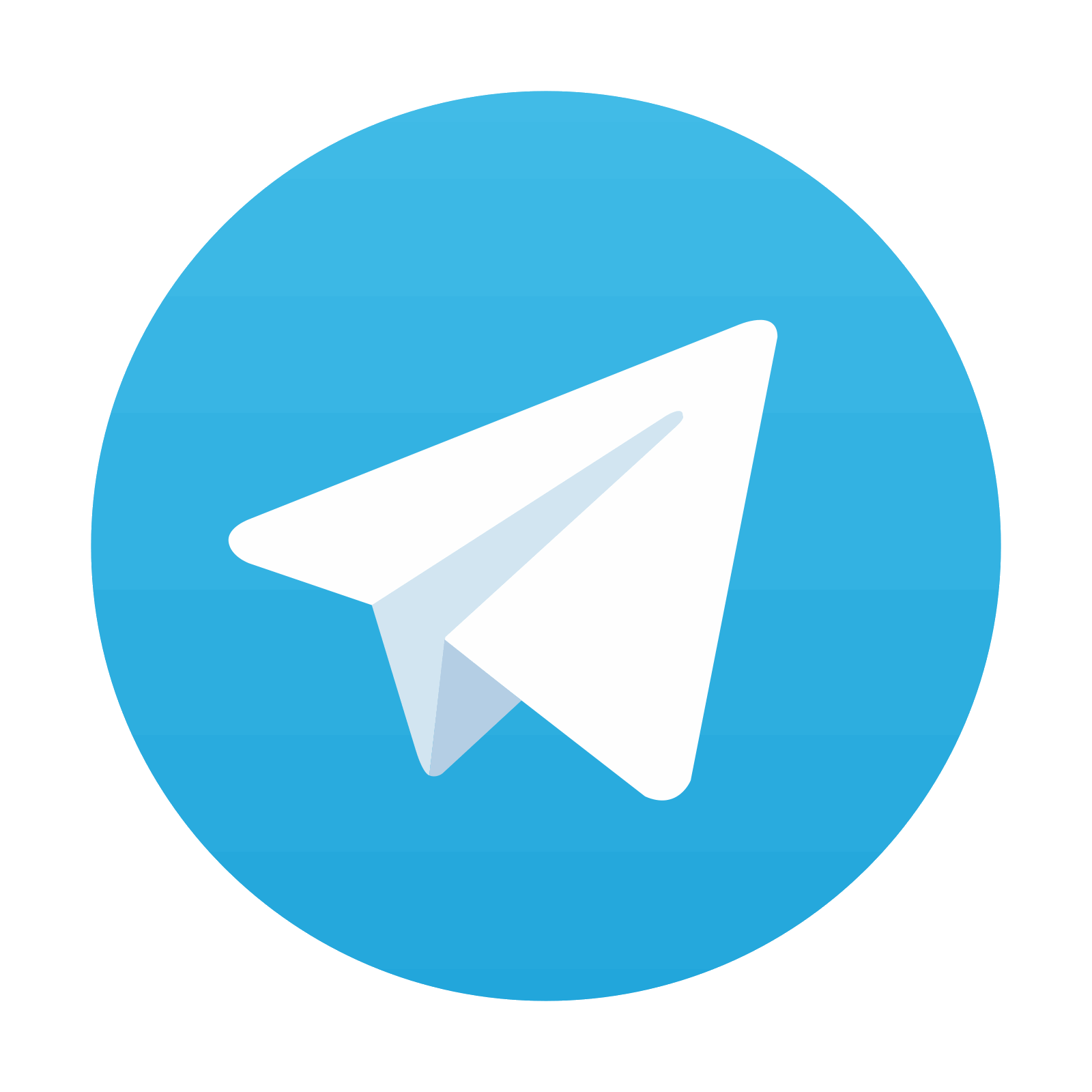
Stay updated, free articles. Join our Telegram channel
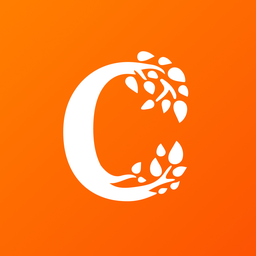
Full access? Get Clinical Tree
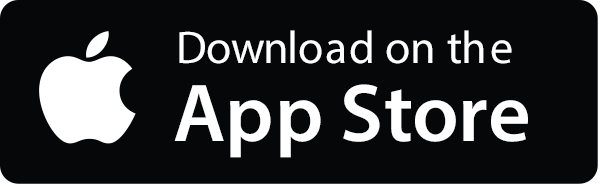
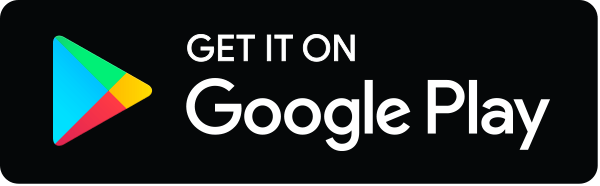