Fig. 20.1
FlaSh. (a) GFPΔC (green) was inserted in-frame into the Shaker K1 channel (black). A point mutation W434F (red circle) was made in the pore of the channel to eliminate ionic current through the sensor. (b) Simultaneous two-electrode voltage-clamp recording and photometry show current and fluorescence changes in response to voltage steps (V) between −60 and 10 mV, in 10 mV increments. Holding potential was −80 mV. The mutated FlaSh exhibits no ionic current. FlaSh fluorescence (F) decreases reversibly in response to membrane depolarizations. Traces are the average of 20 sweeps. Fluorescence scale, 5 % ΔF/F (Modified from Siegel and Isacoff (1997))
FlaSh, Flare (a variant based on a mammalian potassium channel), and two additional first generation FP voltage sensors also based on mammalian channels (VSFP1 and SPARC (Sakai et al. 2001; Ataka and Pieribone 2002)) functioned well in frog oocytes, but they have not been useful in mammalian systems because of poor plasma membrane expression in mammalian cells. No voltage dependent optical signals could be detected in the average of 16 trials using either Flare, VSFP1, or SPARC (Baker et al. 2007). Several strategies to improve membrane expression were tried. Unfortunately, mutagenesis of potential ER retention signals, addition of ER release motifs, and expression in hippocampal neurons that might endogenously express trafficking partners all failed to improve the plasma membrane expression (K. Ray, K. Tomita, Y. Iwamoto, D. Dimitrov, A. Perron, T. Knöpfel, L.B. Cohen, and B.R. Baker, unpublished observations).
3 Second Generation FP Voltage Sensors
The voltage-sensitive phosphatase from the sea squirt, Ciona intestinalis, is a voltage-controlled enzyme consisting of a transmembrane voltage sensor domain and a cytosolic phosphoinositide phosphatase domain (Murata et al. 2005). The voltage sensor domain from this protein was shown to be functional when the enzyme domain was removed (Murata et al. 2005). It was also reported that the phosphatase can exist as a monomer in the plasma membrane (Kohout et al. 2007). This self-contained voltage sensor domain that functioned without additional protein components and without the need for subunit multimerisation was an interesting candidate for a FP-voltage sensor. With the hope of an improvement of plasma membrane targeting in mind, the Thomas Knopfel laboratory explored the use of the Ciona voltage sensor domain (Dimitrov et al. 2007). The CFP and YFP FRET pair from the first generation VSFP1 was fused to the C-terminus of the Ciona voltage sensitive domain. The cytosolic phosphatase domain was replaced by the FRET pair. When expressed in PC12 cells or hippocampal neurons, the second generation VSFP (VSFP2) displayed bright fluorescence and clear targeting to the plasma membrane (Fig. 20.2a) as well as fluorescence changes in response to changes in membrane potential (Dimitrov et al. 2007; Fig. 20.2b). This is a remarkable result. Sensors based on mammalian membrane proteins did not express well in mammalian cells while a sensor based on the sea quirt protein did express well.
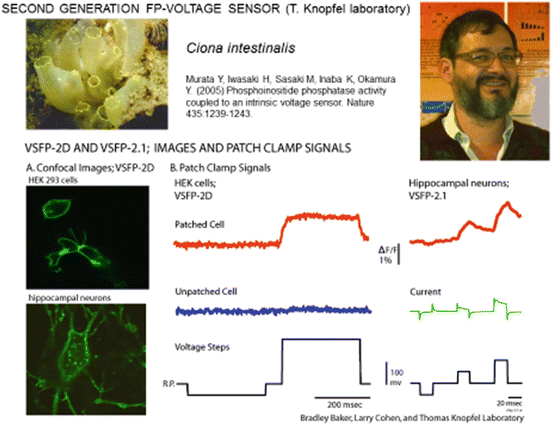
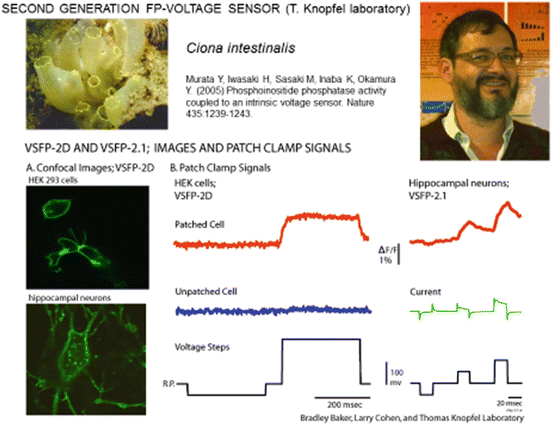
Fig. 20.2
Second generation FP-voltage sensors using the Ciona intenstinalis voltage sensing domain. (A) Images of transfected cultured neurons showing good trafficking to the external membrane of VSFP-2D. (B) Fluorescence signals in response to voltage clamp steps in HEK293 cells and hippocampal neurons. These signals are relatively small (~3 % per 100 mv) and not very fast
The FRET fluorescence change could result from a change in the distance or angle between the two chromophores or from environmental sensitive spectral shifts. In some VSFP2 variants the CFP signal is substantially larger than the YFP signal and/or the two signals have differing kinetics suggesting that non-FRET mechanisms are also occurring (Dimitrov et al. 2007; Lundby et al. 2008). At present we have very limited understanding of the mechanism(s) coupling the voltage dependent structural changes in the sensor domain to the changes in fluorescence of the FPs.
Consistent with ‘gating’ currents measured in Ciona phosphatase, the fluorescence voltage curves for VSFP2 exhibited half maximal activation (V1/2) at potentials far above the physiological range of mammalian neurons. To shift the voltage dependency to a more physiological range, a series of mutational alterations were explored in the putative S4 segment of the Ciona voltage sensor domain. The R217Q mutation resulted in the protein termed VSFP2.1 which had a V1/2 value of ~−70 mV (Dimitrov et al. 2007).
The signal size, ΔF/F, of the second generation VSFP2.1 was ~3 % for a 100 mv depolarization (Dimitrov et al. 2007). Tsutsui et al. (2008) generated and tested an additional 300 Ciona based FP-sensors. One of these, named Mermaid, was said to have a signal size substantially larger than that of previously reported sensors. Mutoh et al. (2009) made a direct comparison of Mermaid and two other Ciona variants, VSFP2.3 and VSFP2.4 and reported that the signal sizes (ΔF/F ~5 %) were similar for all three constructs.
The response time courses of the Ciona constructs were best fit by two exponentials (Dimitrov et al. 2007; Lundby et al. 2008; Villalba-Galea et al. 2009; Tsutsui et al. 2008; Mutoh et al. 2009), with time constants of about 5 and 100 ms. The relative proportion of the faster time constant increased with larger depolarizations.
4 Third Generation FP Voltage Sensors
An accelerating effort has been undertaken to develop sensors with larger and faster signals. There have been three approaches. The first is to make changes in all three components of mosaics like VSFP2 and Mermaid: the voltage sensitive domain, the linker between the Ciona protein and the FP, and the FP itself (selected results are illustrated in Table 20.1). The second is the use of microbial rhodopsins as both the voltage sensor and the fluorescent reporter (Table 20.2). The third is the development of a two component FRET system: an absorbing molecule that resides in the membrane and a membrane bound FP that serves as the FRET partner (Table 20.3). As indicated in the three tables, there has been remarkable recent success; many third generation sensors have larger and faster signals.
Table 20.1
Selected mosaic FP voltage sensors based on the Ciona (or an analogue) voltage sensitive domain
ΔF/F for a 100 mv depolarization (HEK293 cells) | Fast ON tau for a 100 mv depolarization (HEK293 cells) (ms) | Single trial signals (neurons in culture) | Single trial in vivo signals | |
---|---|---|---|---|
Single FP sensors | ||||
ArcLighta | 40 % | 10 | Yes | Yesb,c |
Bongwoorid | 20 % | 8 | Yes | Not tested |
ASAP1e | 17 % | 2 | Yes | Not tested |
Butterfly FRET sensors | ||||
Nabi2.213f | 10 % | 3 | Yes | Not tested |
Chimeric VSFP-Butterfly YRg | not presented | 2 | Not tested | Yes |
Mermaid2h | 20 % | 2 | Yes | Yes |
Table 20.2
Selected FP Voltage sensors based on microbial rhodopsin sensors
Sensor | ΔF/F for a 100 mv depolarization (HEK293 cells) | Fast ON tau for a 100 mv depolarization (HEK293 cells) (ms) | Single trial signals (neurons in culture) | Single trial in vivo signals |
---|---|---|---|---|
Archer1a | 80 %b | 2b | Yes | Yesc |
QuasAr2d | 90 % | <1 | Yes | Not tested |
MacQ-mCitrinee | 20 % | 5 | Yes | Yes |
Table 20.3
A two component FP voltage sensor
Sensor | ΔF/F for a 100 mv depolarization (PC12 cells) | ON tau (PC12 cells) (ms) | Single trial signals (neurons in culture) | Single trial in vivo signals |
---|---|---|---|---|
nVOSa,b | 30 %b | 0.5a | Yesa | Not tested |
4.1 Modifying Mosaic Second Generation Probes Based on the Ciona Voltage Sensitive Domain
Dramatic improvements in signal size and speed have been obtained (Table 20.1). ArcLight signals are about eight times larger than those of VSFP2.3 or Mermaid. The large signals were the results of a spontaneous mutation, A227D, in the FP, super ecliptic pHluorin, together with a reduction in the linker length between the Ciona protein and the FP (Fig. 20.3a–c). Nabi2.213, ASAP1, Chimeric VSFP-Butterfly YR, and Mermaid2 all have fast component time constants of ~2 ms; substantially faster than VSFP2.3 or Mermaid (Table 20.1). The functional properties of new mutations are often unpredictable. Thus the improvements in signal size and kinetics are, in large part, the result of testing many mutations in the three components of the mosaic sensors.
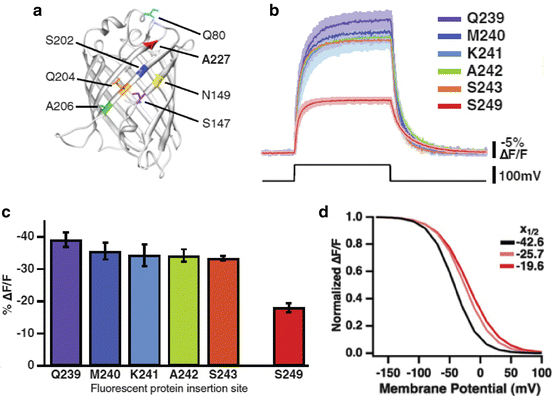
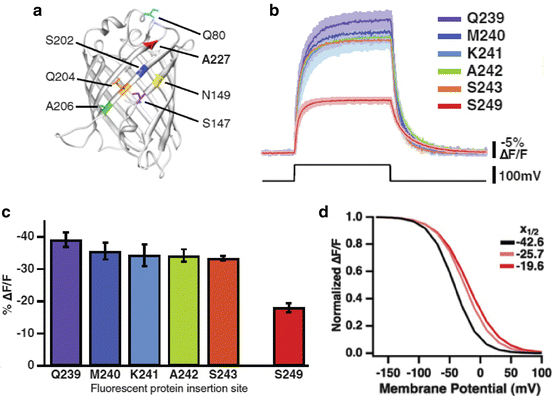
Fig. 20.3
ArcLight, a Ciona based FP voltage sensor with relatively large fluorescence changes. (a) The FP in ArcLight is super ecliptic pHluorin with an A227D mutation. This spontaneous mutation increased the signal size, ΔF/F, from ~1 % to 15 %. The resulting signal is shown in red in (b). (b, c) Shortening the linker resulted in a further twofold increase in the signal size. (d) The fluorescence vs voltage curve for ArcLight (red) is sigmoidal. The V1/2 is −20 mV (Modified from Jin et al. (2012))
Voltage sensitive domain of the phosphatase from other species . Baker et al. (2012) and Han et al. (2013) tested the voltage sensitive domains from species other than Ciona. Signals from chicken, Gallus gallus, and zebrafish, Danio rerio, were relatively large, ΔF/F ~10 %, and fast, tau ~5 ms.
New kinds of FRET probes . In the second generation Mermaid and VSFP, the donor and acceptor FPs were inserted in tandem downstream of the S4 helix. Positioning donor and acceptor FPs at flanking regions of S1-S4 domain was introduced in VSFP Butterfly (Akemann et al. 2012). Three of the probes in Table 20.1, Nabi, Chimeric VSFP-Butterfly YR, and Mermaid2, have butterfly FP locations. Their signal size and kinetics are improved in comparison with the tandem arrangement used in the second generation sensors.
Using circularly permuted FPs . Following the example of GCaMP calcium sensors, probes that incorporated circularly permuted FPs (Baird et al. 1999) into the Ciona voltage sensitive domain were tested but the initial attempts resulted in signals that were small (Gautam et al. 2009: Barnett et al. 2012). St-Pierre et al. (2014) obtained relatively large and fast signals with ASAP1 (Table 20.1) which uses a circularly permutted FP in the extracellular loop between S3 and S4 in the voltage sensitive domain of the chicken phosphatase.
Quantum efficiency . The FP voltage sensors in this group are relatively bright because the FPs are, in part, chosen for their brightness. For example, ArcLight is 50-fold brighter than the microbial rhodopsin sensor QuasAr2 (Adam Cohen, personal communication). In some circumstances (cultured neurons) this difference in brightness can be overcome by using more intense incident illumination for the microbial rhodopsin sensor. In other situations (e.g. in vivo mammalian brain) the high intensity illumination needed for the rhodopsin is likely to cause damage. In addition the signals from a dim sensor can be obscured by the intrinsic fluorescence change and the “intrinsic imaging” blood flow signals.
External membrane expression . The mosaic FP voltage sensors also appear to have relatively selective external membrane expression (Fig. 20.2; Jin et al. 2012; Tsutsui et al. 2013; Storace et al. 2014; Mishina et al. 2014; St-Pierre et al. 2014).
Fluorescence vs membrane potential. All of the sensors in Table 20.1 (except ASAP1) have sigmoidal fluorescence vs voltage relationships (e.g., Fig. 20.3d). The V1/2 can be shifted along the voltage axis by mutations in the transmembrane helices. Thus these FP voltage sensors could be modified to be selectively sensitive to hyperpolarizations, subthreshold depolarizations, or action potentials. Piao et al. (2014) have studied a large number of mutations of the transmembrane helical elements of Bongwoori and related Ciona based sensors.
In vivo measurements. Three of the probes in Table 20.1 have been used to record single trial signals from the mammalian brain. As expected from the arguments given in the Sect. 1, all three recordings were of population signals. Figure 20.4 illustrates results from mouse auditory cortex following embryonic electroporation with Mermaid2 DNA (Tsutsui et al. 2013). Panel c shows the time courses of the donor (blue) and acceptor (orange) signals as well as the ratio signal (black). Both the donor and acceptor signals have heart beat artifacts which are greatly reduced in the ratio signal. Panel b shows a comparison of the signals resulting from a 5 and a 20 kHz acoustic input. The initial responses at 51 ms and 81 ms are in different locations on the cortex.
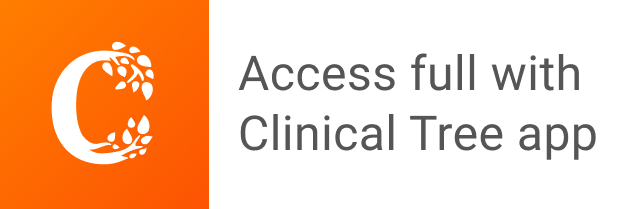