Methods
Energy conduction
Ablation volume
Energy delivery
Imaging guidance
Ablation time (min)
Ablation expense
HIFU
Heat cavitation
Conformal ablation
No tumor size limitation
Transcutaneous
No probe insertion
Ultrasound
MRI
30–120
Expensive device
No probe charge
RFA
Heat
No conformal ablation
Tumor size limitation
Percutaneous
Electrode probe
Ultrasound
CT
10–30
Cheap device
Expensive probe
Laser
Heat
Not Conformal ablation
Tumor size limitation
Percutaneous
Optical fiber
Ultrasound
MRI
25–30
Expensive device
Probe charge
Microwave
Heat
Not Conformal ablation
Tumor size limitation
Percutaneous
Electrode
Antenna
Ultrasound
CT/MRI
20–60
Cheap device
Probe charge
Cryoablation
Cold
Not conformal ablation
Tumor size limitation
Percutaneous
Applicator
Ultrasound
CT
15–30
Cheap device
Expensive applicator
8.2.1 High-Intensity Focused Ultrasound Ablation
Concerning all minimally invasive therapies, HIFU ablation is the only non-invasive approach proposed to date (Kennedy 2005). It employs extracorporeal ultrasound energy to ablate a targeted tumor at depth without any needle insertion. Thus, there is no damage to the skin and overlying tissues. Ultrasound is a high frequency pressure wave. It can be brought to a tight focus at a distance from its source while propagating through tissues. If the concentrated energy is sufficient, energy absorption by the living tissue causes measurable temperature rises (56–100 °C), resulting in coagulation necrosis of the tissue solely within the focal volume (Chaussy et al. 2005). In addition, non-thermal effects, such as cavitation, can induce local tissue destruction due to cavitation-induced high pressures and temperatures (Wu 2006). A single exposure ablation zone (1–3 s) is small, ellipsoidal sized, approximately 1.5 × 15 mm under normal exposure parameters at 1.0 MHz. By placing numerous individual ablation zones side-by-side, conformal confluent ablation volumes of clinically relevant size can be achieved (ter Haar 2007). On the other hand, while HIFU ablation only takes 1–3 s per exposure; the total time can be substantial, longer than other minimally invasive therapies.
8.2.2 Radiofrequency Ablation
RFA uses an electromagnetic energy source with frequencies less than 900 kHz to generate heat (Decadt and Siriwardena 2004). An electrode probe is percutaneously placed into a targeted tumor. Through the probe, there is transmission of low voltage alternating current that creates ionic agitation and heating (Lau and Lai 2009). Ablation temperatures reach 50–100 °C, resulting in the coagulation necrosis of the targeted tumor (Curley 2001). While the tissue surrounding the tip of the probe reaches in excess of 100 °C, it will vaporize and char. This decreases the absorption of the energy, and reduces the ablative size of the surrounding tissue (Goldberg et al. 1996).
8.2.3 Laser Ablation
The term LA is also referred to as laser photocoagulation or laser interstitial thermal therapy (Goldberg et al. 2005). LA employs infrared light energy to produce heat and ablate a targeted cancer. The light energy is transmitted through an optical fiber with a bare tip, and thus induces coagulation necrosis of the targeted tumor while it diffuses through the target (Gough-Palmer and Gedroyc 2008). The Nd-YAG (neodymium:yttrium aluminum garnet) laser with a wavelength of 1064 nm, and diode laser with shorter wavelengths (800–980 nm), are the most widely used devices for laser ablation of solid tumors. They can both induce tissue photocoagulation at low power, or vaporization and cavitation at a higher output. The extent of tissue necrosis is typically limited, dependent on the amount of deposited energy. Thus, multiple fiber applicators are necessary in clinical application for ablation of larger lesions (Sabharwal et al. 2009).
8.2.4 Microwave Ablation
MWA employs electromagnetic energy to ablate a targeted tumor via an electrode-antenna placed within the lesion (Carrafiello et al. 2008). While electromagnetic microwaves (900–3,000 kHz) travel through the tissues, they evoke agitation and vibration of ionic molecules, such as water molecules, within cells. The rapid motion of these ionic molecules causes frictional heating, raising the local temperature range from 60–100 °C in the cellular environment, resulting in tissue coagulation necrosis (Simon et al. 2005). Compared to RFA, microwave ablation can actively heat a much larger area, with less effect on the heat sink, but there is no tissue boiling and charring during ablation procedure (Beland et al. 2007).
8.2.5 Cryoablation
Cryoablation is an alternative technique that uses extreme cold to freeze a targeted tumor in the form of an “ice-ball.” It is one of the oldest ablation methods, with less peri- and post-procedural pain (Rybak 2009). Cryoablation has recently gained an increased interest due to the use of an argon-gas cryotherapy technique, which induces controlled tissue freezing by inserting a percutaneous applicator into a targeted lesion (Dumot and Greenwald 2008). A typical cryoablation session involves a freeze-thaw-freeze cycle. The argon and helium gases are alternately delivered to achieve extra- and intra-cellular ice crystal formation and tissue osmosis. This process causes protein denaturation, cell membrane rupture and cellular death (Babaian et al. 2008).
8.3 Mechanisms of Thermal Ablation and Immune Response
The absorption of physical energy delivered by thermal ablation technique can result in a measurable temperature elevation in living tissue. The thermal effects on tissue are directly dependent on how heat interacts with the tissue. When temperatures are increased to 42–45 °C for a period of 30–60 min, cells become more subject to damage by other agents such as radiotherapy and chemotherapy (Hill and ter Haar 1995). Increasing the temperature can obviously shorten the exposure time for therapeutic effects. If the temperature is increased a few degrees more to 50–52 °C and maintained for 4–6 min, irreversible cellular damage is induced (Thomsen 1991). Between 60 and 100 °C, instantaneous induction of protein coagulation occurs, resulting in the permanent destruction of key mitochondrial enzymes and nucleic acid-histone complexes (Goldberg et al. 2000). Temperatures greater than 105 °C can cause tissue vaporization and carbonization (Goldberg et al. 1996).
Thermal ablation technique is a different therapy to hyperthermia, which has been applied by physical heating technology to elevate targeted regions to temperatures in the 42–45 °C range. This “conventional” hyperthermia usually maintains uniform temperature distributions in a narrow therapeutic range for a period of 30–60 min, and is applied once or twice a week (Diederich and Hynynen 1999). However, the temperature distributions induced in-vivo are usually non-uniform because of tissue cooling by blood flow, and it is extremely difficult to avoid local cold spots that do not reach the required therapeutic temperature level (Lubbe and Bergemann 1994). The efficiency of hyperthermia is highly dependent on the ability to localize and control the successful temperature distributions, which are often influenced by tissue heterogeneities and blood flow. As a result, hyperthermia cannot be used alone in its clinical application, but can be only implemented as an adjuvant method to combine with either radiation therapy or chemotherapy in the treatment of malignant tumors (Dewey 1994). Two types of mechanism are commonly involved to explain the rational for this combined therapy. Heat is a radio-sensitizer that increases radiation damage and prevents subsequent repair. Hyperthermia can also produce biological effects on targeted tumors, including direct cellular toxicity, hypoxia, low pH and indirect blood perfusion deprivation in the tumor (Overgaard 1989).
Thermal ablation can cause direct and indirect damages to a targeted tumor. Direct heat injury occurs during the period of heat deposition, and it is predominately determined by the total energy delivered to the targeted tumor (Nikfarjam et al. 2005a, b, c). Indirect heat injury usually occurs after thermal ablation, which produces a progression in tissue damage. It may involve a balance of several factors, including microvascular damage, cellular apoptosis, Kupffer cell activation and altered cytokine release (Nikfarjam et al. 2005a, b, c). Direct injury is generally better defined than the secondary indirect effects.
8.3.1 Direct Thermal and Non-thermal Effects on Tumor
The effects of thermal ablation on a targeted tumor are determined by increased temperatures, thermal energy deposition, rate of heat removal and the specific thermal sensitivity of the tissue. As the tissue temperature rises, the time required to achieve irreversible cellular damage decreases exponentially. At temperatures between 50 and 55 °C, cellular death occurs instantaneously in cell culture (Wheatley et al. 1989). Protein denaturation, membrane rupture, cell shrinkage, pyknosis and hyperchromasia occur ex-vitro between 60 and 100 °C, leading to immediate coagulation necrosis (Wheatley et al. 1989). Additional to this necrosis, tissue vaporization and boiling occur at temperatures greater than 105 °C. Carbonization, charring and smoke generation occur when the temperature is over 300 °C (Heisterkamp et al. 1997).
In addition, acoustic cavitation, one of mechanical effects induced by HIFU ablation, is the most important non-thermal mechanism for tissue disruption in the ultrasound field (Germer et al. 1998a, b). The presence of small gaseous nuclei within subcellular organelles and tissue fluids are the source of cavitation. These bubbles can expand and contract under influence of the acoustic pressure. During the collapse of bubbles, the acoustic pressure is more than several thousand Pascals, and the temperatures reach several thousand degrees Celsius, resulting in the local destruction of the tissue (Maris and Balibar 2000).
Histological changes are evident in tumor tissue after thermal ablation (Clement 2004). In addition to HIFU ablation, four cellular change zones are described in the liver after thermal ablation as follows: Application, central, transition and reference tissue zones (Ozaki et al. 2003a, b; Germer et al. 1998a, b; Ohno et al. 2001). The application zone is where the heat source contacts the tissue. The central zone immediately surrounds the application zone and consists of damaged tissue. The transition zone contains apparently undamaged tissue, but exhibits signs of subacute hemorrhage. The reference zone refers to normal tissue surrounding the transition zone.
8.3.2 Direct Thermal Effects on Tumor Blood Vessels
Structural and functional changes are directly observed in tumor blood vessels after thermal ablation. These changes are not as well described as the thermal effects on tissues, but they do rely on varying temperatures. At temperatures between 40 and 42 °C, there is no significant change in tumor blood flow after 30–60 min exposure (Ozaki et al. 2003a, b). Beyond 42–44 °C, there is an irreversible decrease in tumor blood flow, with vascular stasis and thrombosis resulting in heat trapping and progressive tissue damage (Emami and Song 1984). While temperatures exceed 60 °C, immediate destruction of tumor microvasculature occurs (Tranberg 2004). It cuts the blood supply to the tumor directly through the cauterization of the tumor feeder vessels, leading to nutrient and oxygen deprivation. Thus, tissue destruction can be enhanced by the damage caused by thermal ablation to tumor blood vessels.
8.3.3 Indirect Effects After Thermal Ablation
Indirect injury is a secondary damage to tissue that progresses after the cessation of thermal ablation stimulus (Muralidharan et al. 2004). It is based on histological evaluation of tissue damage at various time points after thermal ablation (Matsumoto et al. 1992). The full extent of the secondary tissue damage becomes evident 1–7 days after thermal ablation, depending on the model and energy source used (Wiersinga et al. 2003; Benndorf and Bielka 1997). The exact mechanism of this process is still unknown. However, it may represent a balance of several promoting and inhibiting mechanisms, including induction of apoptosis, Kupffer cell activation and cytokine release.
Cellular apoptosis may contribute to progressive tissue injury after thermal ablation. It is well established that apoptosis increases in a temperature-dependent manner, and temperatures between 40 and 45 °C cause inactivation of vital enzymes, thus initiating tumor cell apoptosis (Barry et al. 1990; Hori et al. 1989). Thermal ablation creates a temperature gradient that progressively decreases away from the site of probe insertion. The induction of apoptosis at a distance from the heat source may potentially contribute to the progression of injury. An increased apoptosis rate is observed in the liver 24 h after microwave ablation (Ohno et al. 2001). The stimulation of apoptosis may be directly induced by temperature elevations, alterations in tissue microenvironment and the release of various cytokines after thermal ablation. Kupffer cell activity may be one of the major factors involved in progressive injury after thermal ablation (Heisterkamp et al. 1997). Heat induces Kupffer cell IL-1 (Decker et al. 1989) and tumor necrosis factor-α (TNF-α) (Adams and Hamilton 1984) secretion, which are known to have in-vivo antitumor activity and increase cancer cell apoptosis (Hori et al. 1989). Kupffer cells also induce the production of interferon that augments liver-associated natural killer cell activity (Kirn et al. 1982).
Thermal ablation may induce both regional and systemic production of cytokines through activation of inflammatory cells. Compared with controls, the circulating level of IFN-γ and vascular endothelial growth factor levels markedly increase after RFA (Napoletano et al. 2008; Evrard et al. 2007a, b). The increased level of IL-1 and TNF-α is also observed after RFA (Ali et al. 2005). These cytokines may have direct cytotoxic effects, such as inducing tumor endothelial injury and rendering tumor cells more sensitive to heat-induced damage (Watanabe et al. 1988; Isbert et al. 2004). However, contrasting results are obtained for TNF-α levels in two studies (Evrard et al. 2007a, b; Schell et al. 2002) and IL-1 level in one study (Schell et al. 2002), where levels remain unchanged after thermal ablation. Cryoablation may cause pathophysiological changes, which are similar to those observed after endotoxin administration (Chapman et al. 2000a, b, c; Wudel et al. 2003). These changes cause significant increases in capillary permeability in the lung, leading to secondary injury (Washington et al. 2001). It is generally believed that all alterations may be associated with post-cryosurgery activation in the lungs of the nuclear factor-κB factor and derived cytokines, including TNF-α and macrophage inflammatory protein-2, along with an increase in serum thromboxane levels (Seifert et al. 2002; Sadikot et al. 2002).
8.4 Antitumor Immune Response After Thermal Ablation
8.4.1 HIFU Ablation
As shown in Table 8.2, there is increasing evidence from animal studies that indicate that HIFU may modulate host antitumor immunity after tumor ablation. Yang and colleagues (Yang et al. 1992) used HIFU to treat C1300 neuroblastoma implanted in mouse flanks, followed by the re-challenge of the same tumor cells. A significantly slower growth of re-implanted tumors was observed in these mice compared with the controls. After HIFU treatment, the cytotoxicity of cytotoxic T lymphocytes (CTLs) and the number of activated tumor-specific CTLs was significantly increased in the H22 tumor bearing mice treated with HIFU. Adoptive transfer of the activated lymphocytes could provide better long-term survival and lower metastatic rates in the mice re-challenged by the same tumor cells when compared with sham-HIFU and control groups. This is indicative that HIFU ablation could activate tumor-specific T lymphocytes, thus inducing antitumor cellular immunity in the mice (Xia et al. 2012). Similar results were confirmed in the mice implanted with MC-38 colon adenocarcinoma and melanoma after HIFU ablation.
Table 8.2
Antitumor Immune Response to HIFU Alone in Animal Studies
References | Tumor cell line/model | HIFU parameters | Endpoint | Results | Additional observations |
---|---|---|---|---|---|
Yang et al. (1992) | C1300 Neuroblatoma Ajax (A/J) mice | 4 MHz 550 W/cm2 | Resistance to rechallenge | Significant inhibition of tumor growth in mice treated with curative HIFU compared to the untreated controls | Single & repeated HIFU could prolong survival rates in the tumor bearing mice |
Xia et al. (2012) | H22 Hepatocarcinoma C57BL/6 J mice | 9.5 MHz 5 W 180–240 s | Measurement of CTL cytotoxicity & resistance to rechallenge after adoptive transfer of the activated lymphocytes | Significant increase in CTL cytotoxicity Superior protection after adoptive immunotherapy | Significantly increased number of activated tumor-specific CTLs |
Xing et al. (2008) | B16F10-LucG5 melanoma C57Bl/6 mice | 3.3 MHz | Measurement of CTL cytotoxicity | Significant increase in CTL cytotoxicity in mice treated with curative HIFU compared to the untreated controls | HIFU couldn’t increase the risk of distant metastasis |
Hu et al. (2007) | MC-38 colon adenocarcinoma C57Bl/6 mice | 3.3 MHz | Resistance to rechallenge & cytotoxicity assays of splenic lymphocytes | Superior protection & tumor-specific lymphocyte mediated cytotoxicity after both thermal & mechanical HIFU treatments Antitumor immunity induced by cavitation-based HIFU was stronger compared to thermal HIFU | HIFU could enhance dendritic cell (DC) infiltration in the treated tumor & subsequent migration to draining lymph nodes |
Zhang et al. (2010) | H22 hepatocarcinoma C57BL/6 J | 9.5 MHz 5 W 180–240 s | Resistance to rechallenge Measurement of DC activation & CTL cytotoxicity after immunization with HIFU-generated tumor vaccine | Significant increase in CTL cytotoxicity & DC activation Superior protection in mice immunized with HIFU-generated tumor vaccine when compared with the controls | HIFU treatment alone could enhance CTL cytotoxicity & resistance to rechallenge |
Deng et al. (2010) | H22 Hepatocarcinoma C57BL/6 J mice | 9.5 MHz 5 W 180–240 s | Measurement of DC activation & CTL cytotoxicity Resistance to rechallenge after immunization of DCs loaded with HIFU-treated tumor | Significant increase in DC activation & CTL cytotoxicity with inhibition of tumor growth in mice immunized by DCs loaded with HIFU-treated tumor when compared with the controls | |
Hu et al. (2005) | MC-38 mouse colon adenocarcinoma In-vitro | 1.1 MHz P+ 12/P− 6.7 MPa P+ 31.7/P− 10.7 MPa DC 30 or 3 % 5 s or 30s | Measurement of endogenous danger signals released from HIFU-treated cells Activation of APCs | Release of ATP and HSP-60 from HIFU-treated tumor cells Activation of DCs & macrophages | |
Kruse et al. (2008) | Transgenic reporter mouse for HSP70-Luc2AeGFP | 1.5 MHz 53–353 W/cm2 | Skin HSP-70 expression after 1 s HIFU treatment | Upregulated HSP-70 expression after HIFU treatment | |
Hundt et al. (2007) | Transfected HSP-70-Luc M21 Melanoma NIH-3 T3 mouse fibroma SCCVII mouse squamous cell carcinoma cells in-vitro | 1 MHz 28–179 W/cm2 | HSP-70 expression in tumor cells after either thermal stress or HIFU treatment | Increased HSP-70 expression after both thermal stress & HIFU treatment Higher expression observed at HIFU-induced lower temperatures than thermal stress alone | |
Liu et al. (2010) | B16 melanoma C57BL/6 mice | 3.3 MHz P+19.5/P−7.2 MPa 4 s | Measurement of DC infiltration & maturation in HIFU-treated tumor | Significant increase in local DC infiltration & maturation after HIFU treatment compared to the controls | Sparse-scan HIFU was more effective than dense-scan HIFU in enhancing DC infiltration & maturation in-situ |
Zhou et al. (2007) | H22 hepatocarcinoma Chinese Kun Ming mice | 9.5 MHz 5 W 180–240 s | Resistance to rechallenge after immunization with HIFU-treated tumor vaccine | Significant protection in mice immunized with HIFU-treated tumor compared to heat-treated tumor group Activation of DCs and Macrophages | A significant increase in CD4+ levels & CD4+/CD8+ ratio in both HIFU & thermal groups |
HIFU treatment could also induce an enhanced CTL activity in-vivo, thus providing protection against subsequent tumor re-challenge (Xing et al. 2008). In addition, HIFU could enhance infiltration of dendritic cells (DCs) in the treated tumor and subsequent migration to the draining lymph nodes. Compared to thermal HIFU treatment, antitumor immunity induced by mechanical HIFU treatment (being a pulsed HIFU exposure with no significantly elevated temperature increase in tumor tissue and thermal necrosis) was significantly stronger in terms of DC and CTL activation, and a superior protection against tumor re-challenge was reported (Hu et al. 2007).
After HIFU ablation, large amounts of tumor debris remain in-situ, and the host gradually reabsorbs the debris as the normal process of the healing response. Using a murine hepatocellular carcinoma model, Zhang and colleagues (Zhang et al. 2010) demonstrated that the remaining tumor debris induced by HIFU could be immunogenic, thus an effective vaccine to elicit tumor-specific immune responses. In this study, these included induction of CTL cytotoxic activity, enhanced activation of DCs and protection against lethal tumor challenge in naïve mice. When the tumor debris was loaded with immature DCs, it could significantly induce DC maturation, increase cytotoxicity and CTL TNF-α and IFN-γ secretion, thus initiating a host-specific immune response after H22 challenge in the vaccinated mice (Deng et al. 2010). Immediately after HIFU exposure to MC-38 colon adenocarcinoma cells in-vitro, the release of endogenous danger signals, including HSP60, was observed from the damaged cells. These signals could subsequently activate antigen-presenting cells (APCs), leading to an increased expression of co-stimulatory molecules and enhanced secretion of IL-12 by DCs and TNF-α by macrophages (Hu et al. 2005). In addition, HIFU could upregulate in-vitro and ex-vitro molecular expression of HSP70 (Kruse et al. 2008; Hundt et al. 2007). This is an intracellular molecular chaperone that can enhance tumor cell immunogenicity, resulting in potent cellular immune responses.
The potency of DC infiltration and activation following mechanical lysis and sparse-scan HIFU was much stronger than that from thermal necrosis and dense-scan HIFU exposure, suggesting that optimization of a HIFU ablation strategy may help in enhancing immune responses after treatment (Liu et al. 2010). Heat and acoustic cavitation are two major mechanisms involved in HIFU-induced tissue damage, while cavitation is a HIFU-unique effect when compared with other thermal ablation techniques. It causes membranous organelles to collapse, including mitochondria and endoplasmic reticulum, as well as cell and nuclear membranes. This breaks tumor cells up into small pieces, by which the tumor antigens can remain intact, or it may lead to the exposure of an immunogenic moiety that is normally hidden in tumor antigens. Zhou and colleagues (Zhou et al. 2007) used either heat-exposed or HIFU-treated H22 tumor vaccines to inoculate naïve mice. The vaccination times were four sessions once a week for four consecutive weeks, and each mouse was challenged with H22 tumor cells 1 week after the last vaccination. They found that the HIFU-treated tumor vaccine could significantly inhibit tumor growth and increase survival rates in the vaccinated mice, suggesting that acoustic cavitation could play an important role in stimulating the host antitumor immune system.
Emerging clinical results revealed that systemic cellular immune response was observed in cancer patients after HIFU treatment, as shown in Table 8.3. Rosberger and colleagues (Rosberger et al. 1994) reported five consecutive cases of posterior choroidal melanoma treated with HIFU. Three patients had abnormal, and two patients normal CD4+/CD8+ ratios before treatment. One week after treatment, the ratio in two patients reverted to normal, while another was noted to have a 37 % increase in CD4+ T cells relative to CD8+ cells. Wang and Sun (2002) used multiple-session HIFU to treat 15 patients with late stage pancreatic cancer. Although there was an increase in average numbers of NK cells, T lymphocytes and subsets in ten patients after HIFU treatment, a significant statistical difference was only observed in NK cell activity before and after HIFU treatment (p < 0.05). Wu and colleagues (Wu et al. 2004) observed changes in circulating NK, T lymphocyte and subsets in 16 patients with solid malignancy before and after HIFU treatment. The results showed a significant increase in the CD4+ T lymphocyte population (p < 0.01) and the ratio of CD4+/CD8+ cells (p < 0.05) after HIFU treatment. The abnormal levels of CD3+ lymphocytes returned to normal in two patients, CD4+/CD8+ ratio in three patients, CD19+ lymphocytes in one patient and NK cells in one patient, in comparison to the values in the control group. In addition, serum levels of immunosuppressive cytokines, including VEGF, TGF-β1 and TGF-β2, were significantly decreased in peripheral blood of cancer patients after HIFU treatment, indicating that HIFU may decrease tumor-induced immunosuppression and renew host antitumor immunity (Zhou et al. 2008).
Table 8.3
Antitumor immune response to HIFU alone in clinical studies
Reference | Tumor/nb. patients | HIFU parameters | Endpoint | Results | Additional observations |
---|---|---|---|---|---|
Rosberger et al. (1994) | Choroidal melanoma /5 | 4.6 MHz 2 W/cm2 | Measurement of T cells & subsets in peripheral blood | CD4+/CD8+ ratio reverted to normal after HIFU in 2 of 3 patients with previously abnormal CD4+/CD8+ ratio | 37 % increase in CD4+ relative to CD8+ in the remaining one patient |
Wang and Sun (2002) | Pancreatic cancer/15 | 0.5–1.6 kW 30–80 s | Measurement of T cells, subsets, NK cell activity in peripheral blood | A significant increase in NK cell activity after HIFU treatment | An increase in CD3+, CD4+ & CD4+/ CD8+ ratios in 10 patients after HIFU treatment, but not statistically significant |
Wu et al. (2004) | Osteosarcoma/6 Hepatocarcinoma/5 Renal cell carcinoma/5 | 0.8 MHz 5–20 kW/cm2 | Measurement of circulating NK, T cells & subsets | A significant increase in CD4+ & CD4+/ CD8+ ratios after HIFU treatment | The abnormal levels of CD3+ returned to normal in 2 patients, CD4+/CD8+ ratios in 3 patients, CD19+ in 1 patient & NK cells in 1 patient |
Zhou et al. (2008) | Liver cancer/13 Sarcoma/3 | 0.8 MHz 5–20 kW/cm2 | Measurement of serum immunosuppressive cytokines in peripheral blood | A significant decrease in serum VEGF, TGF-β1 & β-2 levels after HIFU treatment | |
Madersbacher et al. (1998) | Prostate cancer/5 Bladder cancer/4 | 4 MHz 1.26–2.2 kW/cm2 | Measurement of HSP-27 expression in HIFU-treated tumor and prostate tissue | A significant increase of HSP-27 expression after HIFU treatment compared to the controls | |
Kramer et al. (2004) | Prostate cancer/6 | 4 MHz 1.26–2.2 kW/cm2 | Measurement of HSP expression & Th1- & Th2- cytokine release from TILs in HIFU-treated tumor | A significant upregulated expression of HSP-72, HSP-73, glucose GRP-75 & GRP-78 Significant increase in TIL-released IL-2, IFN-γ & TNF-α after HIFU treatment | A significant decrease in TIL-released Th2-cytokines (IL-4, -5, -10) after HIFU treatment |
Breast cancer/23 | 4 MHz 1.26–2.2 kW/cm2 | Measurement of expression of 13 proteins on tumor cells including HSPs | 100 % positive rate of HSP-70 in HIFU-treated cancer cells compared to the control | Varied expressions of ER, PR, CA15-3, VEGF, TGF-β1, TGFβ2, IL-6, IL-10 and EMA in HIFU treated tumor, with no expression of PCNA, MMP-9 and CD44v6 | |
Xu et al. (2009) | Breast cancer/23 | 4 MHz 1.26–2.2 kW/cm2 | Measurement of APC infiltration & activation in HIFU-treated tumor | A significant increase in local infiltration & activation of DCs & macrophages compared to the control | |
Liu et al. (2010) | Breast cancer/23 | 4 MHz 1.26–2.2 kW/cm2 | Measurement of TIL infiltration & activation in HIFU-treated tumor | A significant decrease in tumor-infiltrating CD3+, CD4+, CD8+, CD4+/CD8+, B lymphocytes, NK cells, FasL+, Granzyme+ and perforin+ TILs when compared with the control |
Clinical evidence suggests that HIFU treatment may also enhance local antitumor immunity in cancer patients. Kramer and colleagues (Madersbacher et al. 1998; Kramer et al. 2004) found that HIFU treatment could alter the presentation of tumor antigens in prostate cancer patients, which was most likely to be stimulatory. Histological examination showed significantly upregulated expression of HSP72, HSP73 and glucose regulated protein (GRP) 75 and 78 at the border zone of HIFU treatment in prostate cancer. Heated prostate cancer cells exhibited increased Th1-cytokine (IL-2, IFN-γ, TNF-α) release, but decreased Th2-cytokine (IL-4, -5, -10) release from tumor infiltrating lymphocytes (TIL). The upregulated expression of HSP70 was confirmed in the tumor debris of breast cancer after HIFU ablation (Wu et al. 2007a, b), indicating that HIFU may modify tumor antigenicity to produce a host immune response.
Xu and colleagues (2009) found the number of tumor-infiltrating APCs, including DCs and macrophages, increased significantly along the margin of HIFU-treated human breast cancer, with an increased expression of HLA-DR, CD80 and CD86 molecules. Activated APCs may take up the HSP-tumor peptide complex, which remains in the tumor debris and present the chaperoned peptides directly to tumor-specific T lymphocytes with high efficiency, resulting in potent cellular immune responses against tumor cells after HIFU treatment.
Furthermore, HIFU could induce significant infiltration of TILs in human breast cancer, including CD3+, CD4+, CD8+, B lymphocytes and NK cells. The number of the activated CTLs expressing FasL+, granzyme+ and perforin+ significantly increased in the HIFU-treated tumor, suggesting that specific cellular antitumor immunity could be locally triggered after HIFU treatment (Lu et al. 2010).
8.4.2 Radiofrequency Ablation
With regards to minimally invasive therapies, RFA is only one technique that has been widely used in the clinical management of solid tumors, particularly in hepatocellular carcinoma (HCC). As coagulative necrosis is immediately induced in a targeted tumor after thermal ablation, necrotic cell death can be recognized by the immune system as a result of dangerous events, according to the “danger” model of immunity by Matzinger (Gallucci et al. 1999; Matzinger 2002). It is also accompanied by the release of “danger signals” from the heat-stressed cells, such as acute phase proteins, pro-inflammatory cytokines and heat shock proteins (HSPs), thus developing a temporary inflammatory stress. This stress may be associated with positive processes similar to the healing of injured tissues, but could also lead to the stimulation of tumor growth (Gravante et al. 2009). After RFA treatment, a moderate and temporary systemic inflammatory response has been observed in cancer patients, as demonstrated by the increase in plasma levels of pro-inflammatory cytokines and acute phase reactants (Evrard et al. 2007a, b; Schell et al. 2002; Meredith et al. 2007; Schueller et al. 2003; Fietta et al. 2009).
HSPs are families of highly conserved proteins involved in mechanisms of cell repair. They are intracellular molecular chaperones that physiologically bind tumor peptide antigens and enhance tumor cell immunogenicity (Pockley 2003). APCs take up HSP-tumor peptide complex and present the chaperoned peptides directly to tumor-specific T lymphocytes with high efficiency, resulting in potent cellular immune responses against tumor cells (Todryk et al. 2003). Around the necrotic ablated area, RFA produced sub-lethal injury in the zone of transition that showed apoptosis, and increased HSP70 expression in the liver of normal swine (Schueller et al. 2004). Schueller and colleagues found that there was an increased synthesis and cell surface expression of HSPs (HSP70, 90) after RFA in nude rats bearing human hepatocellular carcinoma (Rai et al. 2005). In addition, large amounts of tumor debris could induce local infiltration of activated DCs, the most potent APC for induction of adaptive immunity against cancer (Melief 2008). Activating signals, including necrotic tumor cells and HSPs, could induce the progression of infiltrating DCs from an immature to a mature stage, resulting in the presentation of tumor antigens by mature DCs to naïve T lymphocytes in a MHC-restricted fashion (Lutz and Schuler 2002). Ali and colleagues demonstrated that a transient function of myeloid DCs could be activated in HCC patients 7–14 days after RFA, with an increased ability to stimulate CD4+ T cells (Ali et al. 2005). Up to 7 % of DCs present in the draining lymph nodes contained tumor antigens in the ablated tumor after RFA. Compared to untreated HCC and normal liver tissue, expression of costimulatory molecules, such as CD80 and CD86, was significantly enhanced by incubation with RFA-treated HCC (den Brok et al. 2006). Similar results were also demonstrated by Zerbini and colleagues (2008) in HCC patients; indicative that local tumor ablation could lead to efficient antigen loading, migration and maturation of APCs, including DCs and monocytes. Direct evidence has recently been found that RFA could induce weak tumor-induced immunity in a murine tumor model harboring APC infiltration and amplification, and that enhanced systemic antitumor T cell immune responses and tumor regression was associated with increased infiltration of DCs after subtotal RF ablation (Dromi et al. 2009). These results suggest that the generation of heat-altered tumor antigens, in combination with the “dangerous signals”, may help to overcome immune tolerance or allergy towards the remaining tumor.
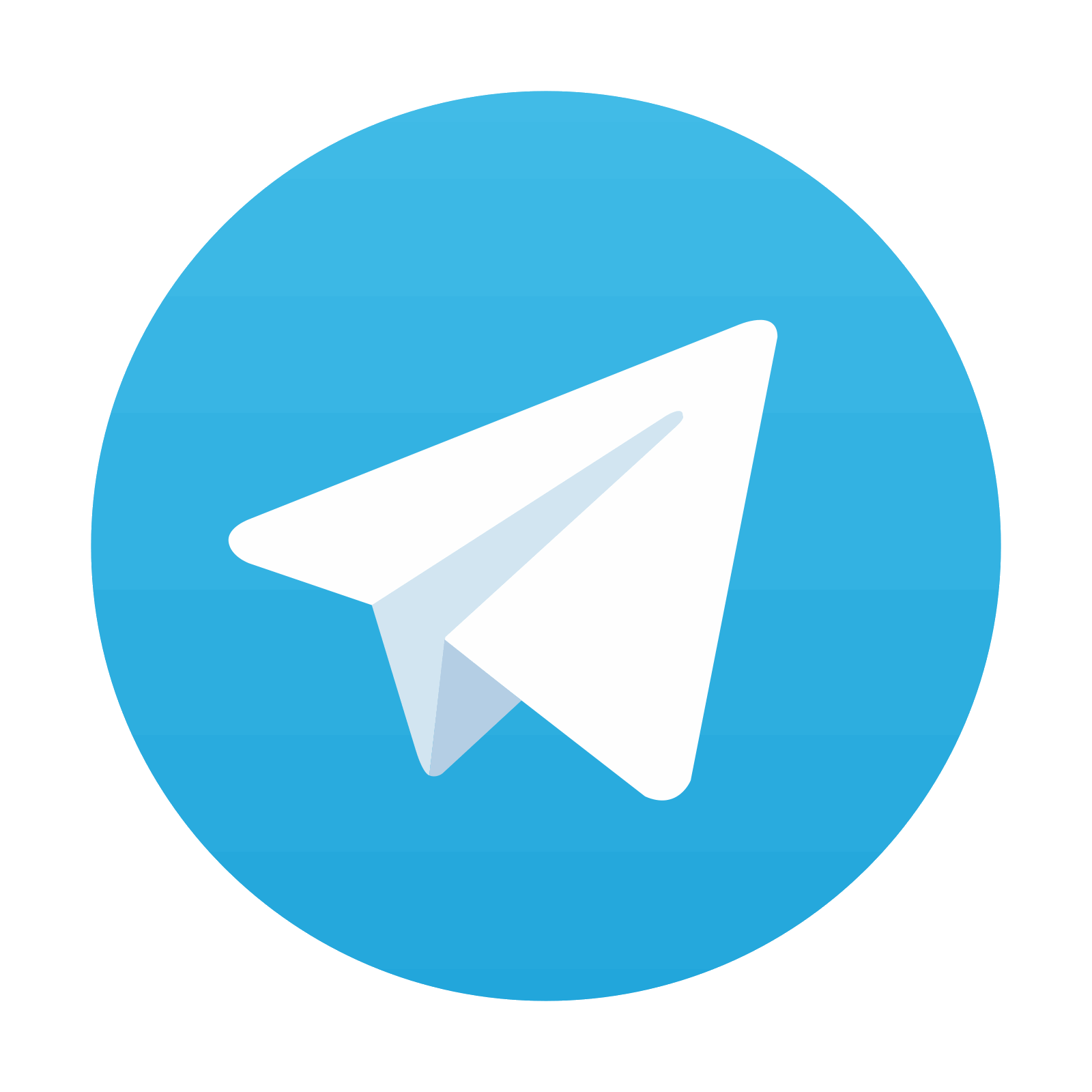
Stay updated, free articles. Join our Telegram channel
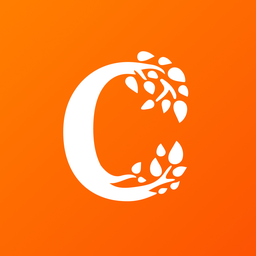
Full access? Get Clinical Tree
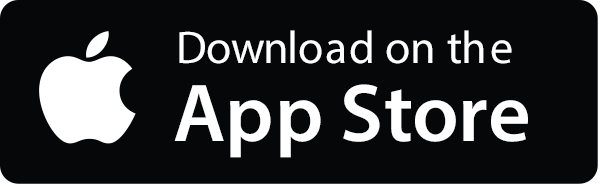
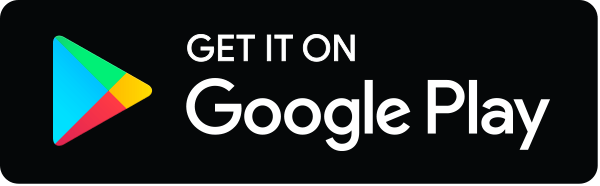