Fig. 59.1
References to guided liver surgery in PubMed and Google Scholar since 1983
Given that the term Image-Guided Neurosurgery appeared in 1989 [1] and the first commercial guidance system appeared in 1993, why did the delay occur in image-guided liver surgery? Two of the biggest reasons were (1) neurosurgery had 40 years of stereotactic frame guidance on which to build, and (2) without a rigid encompassing skull, there were greater challenges in developing a liver guidance system. It is the development of approaches to those challenges which is the central theme of this chapter.
Motivation
Liver cancer is bimodal in distribution with the USA having a different distribution than almost all other countries. In the USA, at present, the biggest group of liver cancer patients is those with metastatic disease from other organ cancers. For example, it is estimated that 142,500 new cases of colorectal cancer will present each year in the USA [2] from which 50 % [3] will develop metastatic liver disease. Liver metastases occur in many patients with other primary malignancies (e.g., breast cancer) as well and are a frequent cause of cancer-related deaths. Unfortunately, it is estimated that there are only 6,000–12,000 patients who are deemed resectable using current techniques [4]. These figures do not include the nearly 19,000 US patients with hepatocellular carcinoma (HCC) and the 3,000 with cholangiocarcinoma (hilar and intrahepatic) with primary intrahepatic malignant tumors [5]. Interestingly, in reviewing the patient base with potentially resectable colorectal metastases, fewer actually undergo resection [6]. This is unexpected since surgical resection remains the most successful with 5-year progression-free outcomes. Surgical outcomes now approach 60 % progression-free survival (PFS), when adjuvant therapies are used with surgical resections [7]. While ablation techniques are improving and there are calls for randomized trials, the success of ablative techniques has occurred in small lesions and the best 5-year outcomes significantly trail resection [8]. The reason that many of these patients do not undergo resection is, for the most part, failure of referral to specialists in the field, perhaps related to the magnitude of the liver resection operations in the setting of metastatic cancer.
The second mode of liver cancer distribution is the non-US market. HCC is associated with cirrhosis in approximately 80 % of patients, usually in the setting of viral hepatitis (hepatitis B (HBV) or hepatitis C (HCV)), alcohol use, or steatohepatitis among other causes. A 2004 epidemiology [9] study predicted more than half a million of new cases of HCC a year, and this study was released before the extent of the Asian hepatitis problem was known. A 2008 study [10] demonstrated that the epidemiology study underestimated the problem as it identified 748,000 cases in 2008. The increased incidence of HBV and HCV will lead to the growth of the HCC problem in the USA [11] while better antiviral treatments and the increasing use of vaccination for HBV may reduce the worldwide occurrence [12, 13].
Liver Organization
The liver has many functional roles but primarily produces important synthetic proteins (e.g., albumin and clotting factors, among others) and is one of the critical sites of metabolic activity. Nutrient absorption from the gastrointestinal tract is cleared on a first-pass basis in the liver via the portal venous system with absorption of glucose and amino acids. In addition, systemic metabolites (e.g., hemoglobin) are cleared from the circulatory system and excreted into bile.
Anatomically, the liver is composed of eight segments originally described by Couinaud (Fig. 59.2).
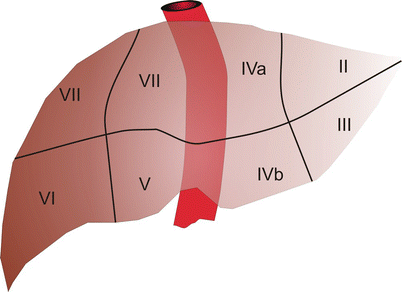
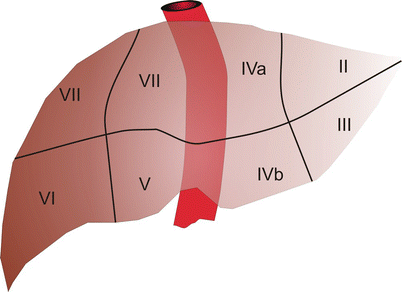
Fig. 59.2
The liver demonstrating the seven of the eight Couinaud segments
Each of these segments contains an individual hepatic arterial, portal venous, and hepatic venous branch. And, in addition, each has its own biliary tract drainage. These components are all necessary for the normal function of a liver segment. Anatomically, segments 1 through 4 comprise the left hemiliver while segments 5 through 8 comprise the right hemiliver. Each hemiliver can be further subdivided into sectors which contain two individual segments (e.g., right anterior sector). Unfortunately, the internal segmental boundaries are not well defined for most segments on the surface of the liver. While preoperative imaging is the primary basis used by surgeons considering liver resection, operative resectional planning has not been conducted in a formalized way and until recently was not an option for most liver surgeons. In this regard, knowledge about segmental boundaries has been poorly defined, and furthermore, the ability to execute planned segmental resections has been limited.
The boundary between the right and left hemiliver is described by Cantlie’s Line, which extends from the gallbladder fossa to the suprahepatic vena cava. This imaginary line runs along the course of the middle hepatic vein and is relatively uniform from patient to patient. In addition, resection of segments 2 and 3 (e.g., left lateral sectionectomy) is defined by the falciform ligament. Other resection plains, however, do not follow such defined boundaries, and in addition, it can be difficult to predict when major vascular structures including a portal venous or hepatic venous branch will be encountered during the course of a given liver resection.
Historical Development of Liver Surgery
Techniques of major hepatic resection have only been developed over the last 20–30 years. Prior to this time, partial hepatectomy was fraught with significant complications, especially major hemorrhage. Developments in preoperative imaging (CT and MR) have enabled surgeons to more accurately plan and predict major intrahepatic structures that would be encountered during a hepatic resection. In addition, dissection tools (i.e., CUSA and water-jet dissection) have enabled surgeons to gain comfort with the parenchymal transection portion of the operative procedure. Additional understanding of the risk of post-resection liver failure has come to be understood and especially the role of the future liver remnant volume (FLR). In addition, surgeons have now gained the ability to impact the future liver remnant volume with portal vein embolization. In this procedure the portal venous branches to the portion of the liver planned for resection are embolized using interventional techniques. This results in progressive atrophy of the intended resected segment with compensatory hypertrophy of the future liver remnant. It has now been demonstrated in a number of studies that the future liver remnant can be hypertrophied on the order of up to 10 % (above baseline) when the right or left major portal venous branch is embolized. Following portal venous embolization, the atrophy/hypertrophy interval usually requires 4–6 weeks of observation. In this regard, operative planning can require careful considerations and may take several months of duration prior to the actual operative procedure. These steps including repeated volumetric assessments are made with contrast-enhanced cross-sectional imaging, adding further to the need for precise, reproducible, and repeatable three-dimensional image planning.
Image-Guided Surgery Approaches
There are a number of techniques that attempt to improve the specificity of surgery, that is, to provide more complete resections while minimizing the damage to surrounding healthy tissue. This includes the use of intraoperative tomographic imaging or the use of intraoperative 2D imaging such as ultrasound or laparoscopy. We leave the intraoperative tomography to other chapters. If one looks at surgical assistance solely by ultrasound or laparoscopy, then it is difficult to say that such techniques provide guidance. Ultrasound provides real-time imaging in two spatial dimensions, so unless you restrict the surgical approach to running along the plane of tissue described by the ultrasound image, then it’s difficult to claim that the ultrasound imaging is “guiding” the resection. In addition, as the resection proceeds, air infiltration into the resection plane prevents additional visualization. Finally, if ultrasound is the sole method of localization of undesired structures such as tumors, the low signal-to-noise ratio of ultrasound imaging remains a challenge since a significant number of liver tumors are natively isoechoic. In addition, underlying steatosis and advanced fibrosis or cirrhosis significantly impair the imaging characteristics with ultrasound. The value of contrast agents and “4D” ultrasound to address these problems is still a matter of active research [14, 15 ].
Minimally invasive techniques have gained wide acceptance and the field continues to grow. The advantages of reduced pain, reduced hospital stay and a faster return to work are undeniable. However, a 2008 “consensus conference” on minimally invasive liver surgery [16] failed to achieve a consensus on either approach or even risk-to-reward and highlighted one of the main challenges of laparoscopic-driven surgery. That challenge is the limited visibility of laparoscopic systems. They provide real-time 2D images of surfaces; however, surgery solely by laparoscopy cannot see into an opaque organ. Therefore, any structures such as blood vessels or tumors buried in the liver parenchyma are invisible to the laparoscope. This prevents the laparoscope from providing guidance of the resection plane.
Neither of these analyses states that ultrasound or laparoscopy cannot be of assistance in liver surgery; rather, it is a narrowing of the topics of concern to this chapter. We propose to focus on the use of preoperatively obtained tomograms to allow the preoperative planning of a surgical resection or ablation and the intraoperative guidance of instruments to execute that plan. Laparoscopy and ultrasound may be used to supplement the data available to the surgeon during the surgery, but such applications are left to the needs and expertise of the surgeon.
There are five requirements for image-guided surgery which may have been described in details in other chapters of this book and can be found here [17, 18]. The five are images (and knowledge of their structure and gray scale), a physical space localization device, a methodology for registering the physical space to the image space, display of surgical position, and correction for peri-procedural changes.
Preoperative imaging for liver tumor detection and localization is influenced by individual medical center expertise and preferences. While standard CT scans are often used in the diagnostic phase, multiphase CT [19], combined CT/PET [20], and MRI sequences with and without contrast enhancement [21] have their proponents. However, the majority of these authors focus on tumor detection or predictive value both before and after therapy. For image-guided surgery, the clear delineation of vascular structures may be as important as visualization of tumor margins.
Multislice CT scans have the demonstrated advantage of being able to capture an entire organ volume in a single breathhold, and the speed of scanning allows relatively easy timing of contrast imaging to separate arterial, portal venous, and hepatic venous structures. MRI has the advantages of inherently superior soft-tissue discrimination and lacks CT’s radiation dose issues. But until techniques like compressed sensing [22] which dramatically speed MR acquisition are commonly available, MR may face the challenge of image blur due to liver motion. Recent forces attempting to push down the cost of health care may also favor CT over present MRI technologies.
Once the image set or sets have been acquired in a manner which provides the surgeon with visualization of the structures of interest, those image sets must be matched to the physical space of the patient. This process, known as a registration, determines the mathematical mapping or transformation between image space and physical space. If there is a single translation and a single rotation for that translation, the registration is referred to as rigid. Nonrigid registrations may also be performed but they are mathematically similar to a deformation correction followed by a rigid registration. We will defer discussion of deformation correction to later in this chapter.
Registration processes have a need for correspondence: points, surfaces, or volumes. In one of the earliest image-guided surgery paper [23], a point-based approach was used, by identifying anatomic points on the CT scan and to homologous points seen in the operating room. The difficulty with this was that biological structures rarely exhibit identifiable “points” and, without the use of extrinsic objects placed before imaging and used as fiducials, the registration quality depends on a combination of imaging quality, surgeon skill at identifying locations in both spaces, and luck.
A consortium of German universities and medical centers approached the problem by combining tracked ultrasound and vascular tomographic images [24–26]. By identifying vascular bifurcations in both the preoperative tomograms and in the tracked and calibrated ultrasound images, these corresponding points can be used as points for both rigid and nonrigid registration. The difficulty of establishing correspondence (which vascular bifurcation is this?) confounds this approach and is an ongoing research area. Recent work [27] from this group is using general intensity information from a 3-dimensional ultrasound to match with the CT information, providing additional clues to resolve correspondence issues.
A research group from two institutions in Bern, Switzerland [28], uses a combination of external and internal points. They perform an initial registration using surface identified points and then localized vessel bifurcations via a tracked ultrasound as landmarks to assess perioperative deformation.
A second approach is to use corresponding surfaces. Those surfaces are the one extracted from the preoperative tomograms and another gathered intraoperatively. There are several challenges in this methodology. First, as known from their initial application in medical imaging [29], surface registrations suffer from a difficulty utilizing surfaces which may cover different expanses of the target in question. Second, such techniques do not have a closed form of solution to match the surfaces and rely on iterative approaches such as that of Besl and McKay [30] and derivatives of that approach. Iterative approaches are rarely exhaustive and may suffer from falling into local minima leading to a poor registration. This is especially true in areas of high rotational symmetry. Third, in the absence of true correspondence, there is a difficulty in assessing the quality of the registration before the surgery starts, and assessment after the surgery begins can provide disastrous negative assessments. Lastly, the concept of correspondence in registration is predicated on the sameness of the surfaces being registered. In liver surgery, the actions on the liver: change in patient pose from scan time to surgery, position in the breathing cycle, liver mobilization, placement of surgical sponges, and the resection itself, may all change the shape of the liver, confounding if not negating the presumption of sameness.
While we have explored point-based registration [31], we found the difficulties of localizing homologous points in both image space and physical space to be too great a challenge. We began using surfaces acquired by dragging a tracked probe over the surface of the liver and implemented a fast version of the ICP algorithm [32]. Training and experience influenced the surface acquisition breadth and coverage. Our initial concept was to use a combination of points and surfaces [33], but we found that once the surgeons began getting complete coverage, the surfaces alone provided the best result [34].
The dependence on the surgeon’s skill and discipline at getting a dense, complete surface plus the inherent surface noise of a handheld probe (digging into the surface or bouncing off the surface) limited the accuracy of the description of the registration surface. Miga et al. [32, 35] introduced the idea of using a tracked laser range scanner to acquire a densely sampled regularly spaced intraoperative surface without using a contact probe for neurosurgery. This was adapted to liver applications [36]. With this device a surface composed of three-dimensional points is determined. A high-resolution full-color photograph is taken at the same time. A calibration process allows the color information to be texture-mapped onto the laser range scan data. A second calibration step allows the LSR field of view to be mapped into the surgical guidance system’s space. An LRS and the liver surface are shown in the figure below (Fig. 59.3).
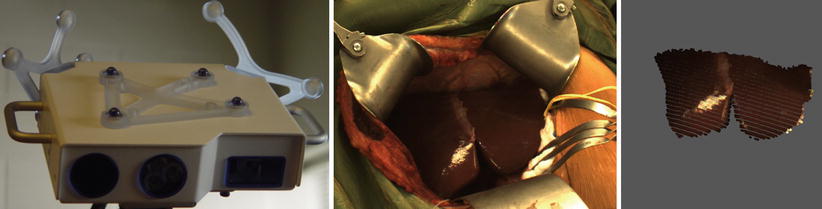
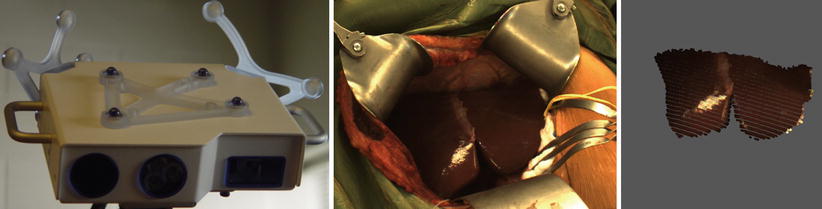
Fig. 59.3
(Left to right) The laser range scanner (LRS), the opened resection cavity, and the laser scan from that cavity
As shown in the Fig. 59.5a, the laser range scan surface and the surface extracted from the CT scan initially are distinct. They are iteratively brought into alignment until some cost function is minimized. That cost function is generally mean surface distance (an unsigned value). Therefore, if two surfaces were to exactly match, half of one surface would lie beneath the second surface and the other half would lie above the second surface. The effect of this sort of algorithm can be seen in Figs. 59.4 and 59.5b.
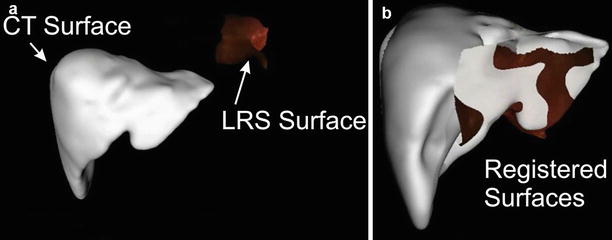
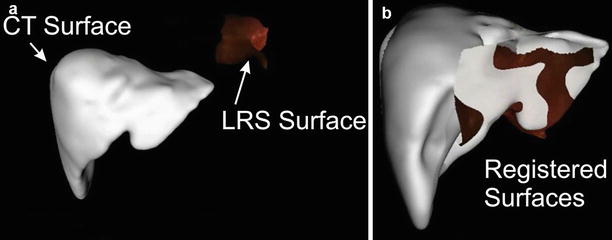
Fig. 59.4
(a) The LRS image and CT-derived liver surface shown prior to registration. (b) The two surfaces after a surface-based registration
With that surface our mean distance between the physical surface and the extracted CT surface was 2.9 mm [37]. While such a metric does not fully quantify the quality of the registration, in the absence of true targets, it is the only available quantitation.
One of the noted drawbacks to an ICP-driven surface registration is that they are sensitive to rotational symmetries and biology tends to create smoothly curved surfaces. Thus, an ICP-driven surface registration can “slide” to incorrect location reducing the robustness of the registration methodology. To address this issue, we elected to capture rough designations of surface features (inferior rims and falciform ligament) in both the image set and the laser range scan images. These “salient features” were weighted with the surface to add information to the registration process [38] (Fig. 59.5).
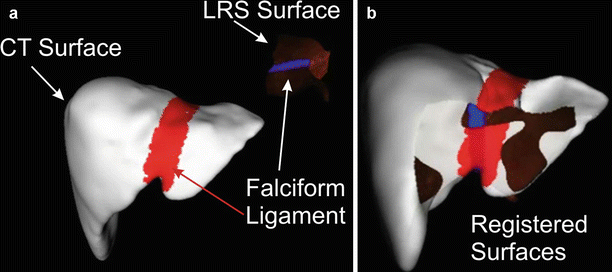
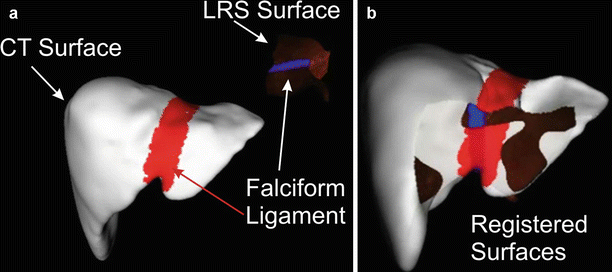
Fig. 59.5
Surface registration of CR-derived surface and an LRS surface with the falciform ligament marked. (a) Prior to registration. (b) After registration
In six sets of clinical data, the localization of observed features had a mean error of 24.15 mm with a standard deviation of 23.7 mm and a median of 18.65 mm. When the “salient feature” registration was applied, the mean error dropped to 3.6 mm, the standard deviation to 1.0 mm, and the median was 3.6 mm.
Because the falciform ligament is roughly linear and a registration could “rotate” around the marked ligament (and in some patients it was difficult to discern the ligament position preoperatively), we use two aspects of the inferior ridge as additional salient features. Figure 59.6 shows those features marked.
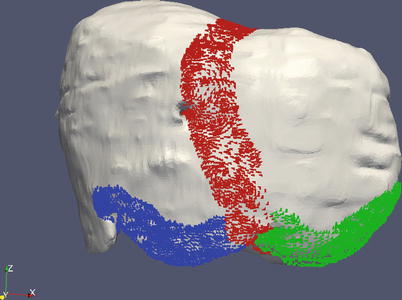
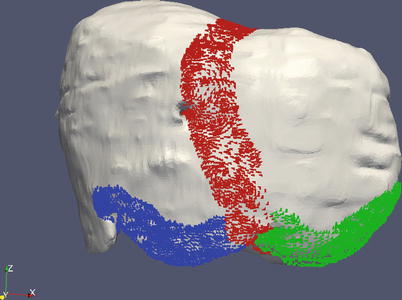
Fig. 59.6
The falciform ligament shown in red, with the inferior ridges marked in green and blue
Beyond the basics of image-guided liver surgery, there have been additional developments. Presurgical planning was pioneered by MeVis (Bremen German). In the MeVis system, the surgeon would submit the preoperative tomograms to a Web-based portal (http://www.mevis.de/loesungen_services_mds.html?&L=1). Through a combination of automatic segmentation and hand refinement, the surgeon would receive back a segmented, annotated set of scans with suggested surgical planes and some volumetric calculations such as estimated residual liver volume [39]. Research work coming from MeVis has begun to provide an additional mapping that of the surgical risk associated with given areas [40] (Fig. 59.7).