Image-guided Stereotactic Radiosurgery and Stereotactic Body Radiation Therapy
Timothy D. Solberg
Brian Kavanagh
Paul M. Medin
PRINCIPLES OF STEREOTACTIC RADIOSURGERY AND STEREOTACTIC BODY RADIATION THERAPY
EVOLUTION OF STEREOTACTIC RADIOSURGERY
The Swedish neurosurgeon Lars Leksell is widely credited as a leading pioneer of stereotactic radiosurgery (SRS) for cranial neoplasms, having initiated work in this field more than 50 years ago.1 Leksell and his colleagues broke from the prevailing conventional wisdom about radiation treatment schedules and created a new paradigm whereby specialized technology was applied to irradiate intracranial tumors to very high doses in a single fraction. It was recognized, of course, that great care must be taken to minimize the amount of normal brain tissue that receives a high radiation dose to minimize toxicity.
In the ensuing half century, SRS has been well studied through extensive collaborations between radiation oncologists and neurosurgeons. SRS has been refined into an important part of the treatment of brain metastases, cerebral vascular malformations, trigeminal neuralgia, and selected primary brain tumors and functional disorders, to name just a few common indications. Modern cranial SRS can be performed noninvasively yet with an extremely high degree of accuracy and on an outpatient basis. New developments in tumor targeting and patient repositioning technology have also allowed for the extension of SRS to lesions outside the central nervous system in close proximity to the spinal cord, where similar concerns about limiting dose to normal tissues apply.
Evolution of Stereotactic Body Radiation Therapy
The success of cranial SRS as an efficient, potent means of local tumor treatment eventually prompted several groups to evaluate analogous strategies of high-dose-per-fraction treatment to extracranial tumors in a variety of sites away from the nervous system. Very much influenced by Leksell’s use of a rigid frame to stabilize the head during cranial SRS, in the early 1990s, fellow Swedes Ingmar Lax and Henric Blomgren constructed a body frame that could comfortably and reproducibly immobilize a patient as well as dampen breathingrelated internal organ motion.2,3 Around the same time, Japanese investigators also began to explore the administration of SRS-like treatment to extracranial sites.4,5 Not long thereafter, enthusiasm for the treatments now known as stereotactic body radiation therapy (SBRT) spread to other centers in Europe, Asia, and North America. The early history of SBRT has been detailed elsewhere.6
Prospective studies of SBRT completed to date have included dose-escalation studies of the treatment of lung and liver metastases,7,8 as well as a Radiation Therapy Oncology Group (RTOG) study of SBRT as treatment for medically inoperable non-small-cell lung cancer (R. Timmerman, Principal Investigator). The Japanese Cooperative Oncology Group and numerous North American and European institutions have also either completed or initiated a variety of studies aimed at improving our understanding of the proper role of SBRT in a broad range of clinical settings.
IMAGE GUIDANCE IN STEREOTACTIC RADIOSURGERY AND STEREOTACTIC BODY RADIATION THERAPY
Fiducial-based Stereotactic Localization
Accuracy and precision in target and dose localization are the hallmarks of the stereotactic technique, and in evaluating image-guided SRS and SBRT techniques, it is instructive to briefly review conventional localization methodologies. Accuracy and precision have been commonly achieved by means of a stereotactic frame, rigidly affixed to a patient’s skull, from which a three-dimensional (3D) coordinate system may be defined. Through a two-dimensional (2D) or 3D imaging procedure, the position of an internal anatomic target can be determined within the external frame of reference (Fig. 6.1). A mechanical device, for example an outer plastic box-like shell, is subsequently attached to the rigid head frame and used to register the patient relative to the radiation source (Fig. 6.2).
A similar paradigm can be used for localization of body targets; although in the absence of rigid anatomy, the “frame” no longer ensures reproducibility between imaging and treatment sessions. The 1990s saw the advent of these fiducial marker-based approaches for extracranial localization. A methodology for radiosurgery of targets involving and adjacent to the spine was described by Hamilton and Lulu9 and Hamilton et al.10 The system consisted of a shallow rigid box, with lateral dimensions compatible with computed tomography (CT) imaging. Patients were placed within the box in a prone position, and, under anesthesia, small clamps were attached to one or two spinous processes adjacent to the intended target. These clamps were rigidly attached to two semicircular metal arches secured to the box. The stereotactic space was defined relative to a small radiopaque sphere using the coordinates system of the CT scanner. Imaging, planning, and treatment were performed in a single setting with the patient rigidly fixed for the duration of the procedure. The authors reported localization uncertainties of 2.0 mm in a worst case scenario. This prototype spinal system was subsequently used in the treatment of nine patients.9, 10, 11 Doses delivered were understandably conservative, ranging from 8 to 10 Gy, with distributions constructed in such a way that no portion of the spinal cords received more than 3 Gy. An attempt to commercially market the “Arizona” spinal radiosurgery system proved unsuccessful.
Medin et al.12 proposed a minimally invasive localization technology that allowed for high-dose, single-fraction irradiation of soft tissue or bony tumors near the spine. Under local anesthesia, three small radiopaque markers were permanently affixed within the vertebral and spinous processes. The implanted fiducials were localized on biplanar radiographs obtained at the time of the planning CT. Both imaging procedures used an external localization box from which a coordinate system was established (Fig. 6.3). At the time of treatment, biplanar radiographs were repeated, the implanted fiducials were identified, and the isocenter position was calculated based on the geometric relationship between the target and implanted markers obtained at the time of CT imaging. In this manner, accurate target localization could be performed despite the fact that (a) the patient had moved from the time of the initial CT and (b) the target could not be directly visualized in the treatment room. In phantoms specially constructed to evaluate overall system accuracy, the worst case targeting error observed was 1.17 mm. The methodology was subsequently evaluated in a swine model. Results indicated that (a) implanted markers were readily distinguishable from normal anatomy on radiographs; (b) markers did not move relative to the intended target or to one another; (c) with the swine in a supine position, markers did not move with normal or forced respiration; and (d) there was little loss in targeting accuracy when markers
associated with one vertebra were used to target adjacent vertebrae.
associated with one vertebra were used to target adjacent vertebrae.
![]() Figure 6.4. The Stereotactic Body Frame (Elekta AB, Stockholm, Sweden) allows stereotactic localization to be performed in a noninvasive manner. (Courtesy of Elekta AB.) |
Concurrent with the earlier work of Hamilton et al.10,11 in Arizona, a group from the Karolinska Hospital in Stockholm, Sweden, had developed a methodology for SBRT localization that, due largely its noninvasive nature, has found broad clinical acceptance in the intervening years.2,3 The system consisted of an immobilization box with embedded CT fiducials and a device for compressing the chest to limit respiratory motion (Fig. 6.4). Localization accuracy was limited to “5 to 8 mm in 90% of setups,” due largely to difficulty in reproducing the patient’s position within the box between imaging and treatment sessions.
A device similar to the stereotactic body frame was constructed by Yenice et al.13 to facilitate SBRT localization. To facilitate improved reproducibility, the patient was set up initially in a standing position, after which the frame and patient were tilted backwards into a horizontal treatment position. The system was also designed to facilitate daily CT imaging, performed just prior to each treatment. The authors were able to demonstrate a localization accuracy of within 1 mm (1σ) in any direction. Daily CT was eventually replaced with localization based on electronic portal imaging, with little loss of targeting accuracy.14
Two groups have combined in-room CT imaging with linear accelerator (linac) delivery for stereotactic irradiation of intra- and extracranial targets (Fig. 6.5). Uematsu et al.15 reported on the treatment of eight patients with primary or metastatic brain tumors. Immobilization was performed using conventional head masks coupled with a dental impression. Localization was achieved by aligning the target to the axis of the CT gantry, marking the corresponding axes with small metallic balls, and subsequently aligning the metallic balls to the lasers of the linac. Phantom studies showed localization
uncertainty on the order of 1 mm. Subsequently, the system has been used extensively for stereotactic targeting of extracranial tumors.4,16
uncertainty on the order of 1 mm. Subsequently, the system has been used extensively for stereotactic targeting of extracranial tumors.4,16
![]() Figure 6.5. Computed tomography (CT) scanner mounted on rails to facilitate stereotactic localization. (Courtesy of Medical College of Wisconsin, Milwaukee, WI.) |
A system combining in-room CT with fiducial-based localization for spinal radiosurgery has also been described by Shiu el al.17 Patients were immobilized in a full-body stereotactic frame and received localization/verification CT scans immediately prior to treatment. This was facilitated by a CT on rails installed in the treatment room. With daily CT imaging, the authors determined that the overall deviation from intended isocenter was within 1 mm for each treatment. Capabilities were later developed to facilitate automated registration of digitally reconstructed radiographs (DRRs) generated from the pretreatment CT scans to DRRs generated from the planning CT.18
Image-guided Stereotactic Localization
Targets outside the skull are not readily amenable to fixation using rigid frames; therefore, image guidance is a prerequisite for extracranial radiosurgery (single-fraction delivery) and SBRT (multiple-fraction delivery). As with frame-based radiosurgery, “frameless” technologies were initially developed to facilitate surgical applications. The first reference depicting frameless capabilities was published by Roberts et al.,19 who described a method for registering CT data with an operating microscope for neurosurgical applications. Subsequent investigators refined this approach,20, 21, 22 and frameless neuronavigation is now commonplace.
![]() Figure 6.6. Principles of stereophotogrammetry. If the position and projection angles of the cameras are known, the location of any object in three dimensions can be determined. |
STEREOPHOTOGRAMMETRIC METHODS OF STEREOTACTIC LOCALIZATION.
Stereophotogrammetry is the general term applied to the science of 3D measurement from two or more overlapping 2D images. By obtaining pictures from at least two different locations and measuring the same target in each picture, a “line of sight” is developed from each camera location to the target. If the camera location and direction are known, the lines can be mathematically intersected to produce the 3D coordinates of each targeted point.
Stereophotogrammetry has found application in many fields, including human growth and motion analysis,23, 24, 25 joint repair and prosthesis fabrication,26 computer-aided analysis of facial expressions,27 and the study of ocular disorders.28 Application of x-ray imaging in stereophotogrammetric analysis (also known as roentgen stereophotogrammetry) has been described by Selvik23 and Johnsson et al.29 (Fig. 6.6).
Image-guided Radiation Therapy Techniques in Stereotactic Radiosurgery and Stereotactic Body Radiation Therapy
NON-X-RAY METHODS OF STEREOTACTIC LOCALIZATION.
The use of stereophotogrammetric techniques for localization of patients undergoing radiation therapy was first described by Schlegel et al.30 and Menke et al.31 They used video stereophotogrammetry as a means of evaluating the repositioning accuracy of a specially designed head holder for fractionated radiotherapy. Shortly thereafter, Bova et al.32 adopted the methodology for cranial radiosurgery. The stereophotogrammetric method was sensitive enough to detect 0.05-mm deflections in a radiosurgery head holder.
Subsequent investigators have implemented infrared (IR) stereophotogrammetry for extracranial localization. Wang et al.33 described a method in which passive IR-reflecting spheres were affixed to the chest and/or abdomen of radiotherapy patients (ExacTrac; BrainLAB AG, Feldkirchen, Germany). Phantom studies demonstrated that the position of each IRreflecting sphere could be determined to < 0.3 mm, although CT-based target localization introduced additional uncertainties
on the order of 3 mm at the 95% confidence level. Ultimately, issues of marker reproducibility and patient motion led the authors to conclude that the accuracy of surface-based IR techniques was inadequate for stereotactic applications.
on the order of 3 mm at the 95% confidence level. Ultimately, issues of marker reproducibility and patient motion led the authors to conclude that the accuracy of surface-based IR techniques was inadequate for stereotactic applications.
Working with investigators at the University of Iowa, Bova and colleagues subsequently coupled their IR-based navigation system with ultrasound image guidance to facilitate targeting of paraspinal tumors.34 System applicability was limited to soft tissue tumors located on the dorsal aspect of the spinal column; disease involving the bony vertebrae, the most common site for metastatic spread, could not be localized due to inherent limitations of ultrasound imaging. The authors subsequently described the treatment of a single patient presenting with a recurrent metastatic squamous cell carcinoma at the level of T11; a dose of 15 Gy was delivered to the 80% isodose line. Bayouth et al.35 subsequently coupled the IR-ultrasound system with a specially designed linac to facilitate cranial and extracranial stereotactic applications.
In a similar manner, Fuss et al.36 used stereotactic ultrasound (BAT; Best Medical International, Inc., Springfield, VA) to target malignancies of the upper abdomen. Due to the challenge of visualizing many of these tumors directly on ultrasound, the authors described the use of adjacent vascular structures as surrogates for target position. They reported that the technique was useful in 95.8% of setups, a significant improvement from traditional ultrasound methodologies. Despite these advances, ultrasound imaging remains challenging in the vast majority of tumor sites, and the use of stereotactic ultrasound is now largely restricted to applications in prostate cancer.37, 38, 39
In 1999, the group at the University of Chicago developed a video-based system for patient positioning.40 The system used two charge-coupled device (CCD) cameras to display real-time subtraction images for analysis of misalignment of head and neck patients. The authors showed that uncertainty could be significantly reduced (from 1σ of 5 to 7 mm to 1σ of 1 to 3 mm) if the system was used for online setup correction. More recently, optical systems have seen a resurgence in interest with the emergence of a commercial technology that uses optical techniques for real-time 3D surface tracking (AlignRT; VisionRT Ltd., London, United Kingdom). In a study using anthropomorphic phantoms, submillimeter differences were observed in the distance between an optical surface model and CT-derived surface topology.41 Although most clinical applications have focused on partial breast irradiation, a group in the United Kingdom has adopted the system for cranial stereotactic radiotherapy (SRT).42 Results suggest that the system provides accuracy comparable with conventional SRT methodologies. Furthermore, localization can be performed in a matter of a few seconds.
ORTHOGONAL KILOVOLTAGE LOCALIZATION.
The principles of stereophotogrammetry can be readily extended to x-ray imaging for direct visualization of internal anatomic structures, with the accuracy necessary for stereotactic applications. Presently there are two commercial systems with x-ray stereophotogrammetry capabilities that are tightly coupled to SRS/SBRT delivery systems—CyberKnife (Accuray Inc., Sunnyvale, Calif) and Novalis (BrainLAB AG, Feldkirchen, Germany). CyberKnife is a robotic radiosurgery system originally designed to treat cranial tumors without a stereotactic head frame.43, 44, 45, 46 The CyberKnife consists of a 6-MeV X-band linac attached to a robotic arm that can move about the patient with six degrees of freedom, coupled with two ceiling-mounted diagnostic x-ray units projecting through the patient to two opposing amorphous silicon detectors recessed within the treatment room floor (Fig. 6.7). The biplanar imaging system provides the capabilities for frameless SRS,47 and because the imaging system is permanently mounted in the treatment room, targeting can be performed without the need for additional “localization boxes.”
Initial CyberKnife applications were for the treatment of cranial disease, benign as well as malignant, treated in a single fraction or multiple fractions.46 However, the integrated image guidance system used by the CyberKnife also makes it suitable for stereotactic irradiation of extracranial tumors. Murphy et al.48 have described modifications to the original CyberKnife to facilitate stereotactic irradiation of spinal and other tumors adjacent to rigid bony anatomy. For the cervical spine, image registration based on bony anatomy is performed between in-room images and DRRs obtained from the planning CT. In the thoracic and lumber spine, however, superposition of bony anatomy can make image-based registration within oblique projections difficult. For these anatomic sites, the authors implant fiducial markers in bony spine adjacent to the target of interest. The fiducial markers are readily localized on x-ray images from which the target position can subsequently be determined. In contrast to the methodology described by Medin et al.,12 markers were inserted in the vertebral bodies (as opposed to the spinous and vertebral processes), and the reference marker locations were derived from CT (as opposed to radiographs). Clinical applications of CyberKnife technology have grown rapidly, and many investigators have now reported on their clinical experience in spine,49, 50, 51, 52, 53, 54, 55 lung,56, 57, 58, 59 liver,60 pancreas,48,61,62 and other extracranial sites. Schweikard et al.63,64 have reviewed the clinical applications in SBRT.
Schweikard et al.65 described the use of the CyberKnife system to compensate for respiratory motion by combining IR tracking of surface markers with coordinated “robotic” motion of the linac component during dose delivery. The motion of the accelerator component is based on the expected relationship between the surface markers and the internal target. A number of subsequent investigators have reported on both technical aspects48,66, 67, 68 and clinical experience58,59,69 using the CyberKnife.
The Novalis is a commercial system introduced in 1997 for image-guided SRS and SBRT applications. The beam delivery system consists of a 6-MeV linac with a micro-multileaf collimator (mMLC) that is mounted permanently to the linac. The unit is capable of several methods of radiosurgery delivery: conventional circular arc radiosurgery; conformal radiosurgery using multiple static shaped beams; dynamic radiosurgery, in which arc delivery is combined with dynamic mMLC field shaping; and intensity-modulated radiotherapy (IMRT). Dosimetric characteristics of the Novalis unit have been described by Yin et al.70
The Novalis system incorporates an IR component to facilitate patient setup and allow for patient position monitoring and also a stereoscopic x-ray component for localization of extracranial targets (Fig. 6.8). The IR component includes two ceiling-mounted cameras that detect the 3D positions of IR-reflective markers placed on the surface of the patient. The IR signal is continuously updated every 50 milliseconds. The kilovoltage (kV) x-ray component consists of two floor-mounted x-ray tubes and two opposing amorphous silicon (aSi) flat panel detectors mounted to the ceiling. Each x-ray tube/detector pair is configured to image through the linac isocenter with a coronal field of view of approximately 18 cm in both the superior-inferior and left-right directions at isocenter. The x-ray localization system can be operated in two modes: matching of implanted radiopaque markers in a manner similar to that of the CyberKnife, and automated registration of x-ray and DRRs using an iterative edge matching algorithm. Comprehensive evaluations of targeting accuracy have been reported by Yan et al.71
With integrated IR and x-ray imaging and delivery capabilities, the Novalis is well suited for advanced treatments that account for respiratory motion. Solberg et al.72 described modifications to the Novalis to allow delivery under gated operation. The ExacTrac (IR) system was used to generate a respiratory signal and trigger the MHOLDOFF/status bit on the console backplane, generating an initial position (IPSN) interlock on the Novalis controller. Hugo et al.73 subsequently performed a systematic dosimetric analysis of dynamic delivery under gated operation. Verellen et al.74 successfully integrated the kV component for image-guided verification of gated operation. Subsequent investigations by Tenn et al.,75 Jin and Yin,76 and Wink et al.77 specifically evaluated effects of respiration amplitude, size of gating window, and latency issues in imaging and beam delivery on localization and gating accuracy. Medin and Verellen.78 have described the Novalis system in detail and reviewed the clinical applications in SRS and SBRT.
As with the CyberKnife, cranial indications were the first clinical applications of the Novalis.79, 80, 81, 82, 83, 84, 85, 86, 87 This was soon followed by interest in using the device in the treatment of extracranial disease. The group at Henry Ford Hospital were pioneers in the use of Novalis for spinal radiosurgery.88, 89, 90 Several groups have reported on the use of the Novalis for SBRT of lung tumors.7,86,91,92 A summary of the Novalis SBRT experience has been published by Teh et al.93
VOLUME-GUIDED LOCALIZATION.
In-room volumetric x-ray guidance, now becoming commonplace in radiotherapy, has been extended to SRS and SBRT applications. Several technologies are capable of producing near real-time 3D images in the treatment room, including conventional kV CT, megavoltage (MV) CT (i.e., tomotherapy), and kV and MV cone beam CT. The inclusion of a conventional CT scanner within the treatment room is generally accomplished in the so-called “CT on rails” format, which requires sliding the patient treatment couch back and forth between image acquisition and treatment delivery positions. Discussed earlier in this chapter, the application of this technique for either SRS or SBRT is still dependent on external fiducials to translate the CT 3D spatial coordinates to the treatment machine’s 3D spatial coordinates and not purely on image guidance.
Chang et al.94 evaluated the accuracy of kV cone beam localization relative to fiducial-based stereotactic targeting. In phantom studies, an uncertainty in the cone beam CT setup procedure of 1.34 ± 0.33 mm was observed. The investigators concluded that localization based on cone beam CT image guidance was equivalent to that of currently used frame-based SRS systems. Létourneau et al.95 have developed a phantom for end-to-end dosimetric and geometric accuracy testing of cone beam image guidance radiosurgery-type applications. To evaluate their methodology, a treatment plan was designed for single-fraction radiosurgery of a spinal target. Image-guided setup was performed, and the phantom was irradiated according to the treatment plan; 97.1% ± 1.5% of measurement points were within 3% of the calculated dose or within 2 mm of distance to agreement.
Two groups from Germany have successfully implemented kV cone beam CT localization for intracranial radiosurgery
and extracranial SBRT treatments. Boda-Heggemann et al.96 used volumetric kV imaging to assess the positioning accuracy and reproducibility in 21 patients undergoing cranial or head and neck irradiation. Automatic 3D-3D matching was used to register cone beam images to the planning CT. Although the study addressed only conventional versus cone beam localization (i.e., there was no absolute reference on which to judge cone beam localization itself), the authors nevertheless concluded that their experience supported a paradigm shift to purely image-guided setup for all intracranial precision radiotherapy procedures. Subsequently, Guckenberger et al.97 used kV cone beam CT for localization of patients receiving SRS for the treatment of brain metastases. They concluded that frameless radiosurgery based on image guidance with registration of the bony anatomy could be performed accurately and efficiently.
and extracranial SBRT treatments. Boda-Heggemann et al.96 used volumetric kV imaging to assess the positioning accuracy and reproducibility in 21 patients undergoing cranial or head and neck irradiation. Automatic 3D-3D matching was used to register cone beam images to the planning CT. Although the study addressed only conventional versus cone beam localization (i.e., there was no absolute reference on which to judge cone beam localization itself), the authors nevertheless concluded that their experience supported a paradigm shift to purely image-guided setup for all intracranial precision radiotherapy procedures. Subsequently, Guckenberger et al.97 used kV cone beam CT for localization of patients receiving SRS for the treatment of brain metastases. They concluded that frameless radiosurgery based on image guidance with registration of the bony anatomy could be performed accurately and efficiently.
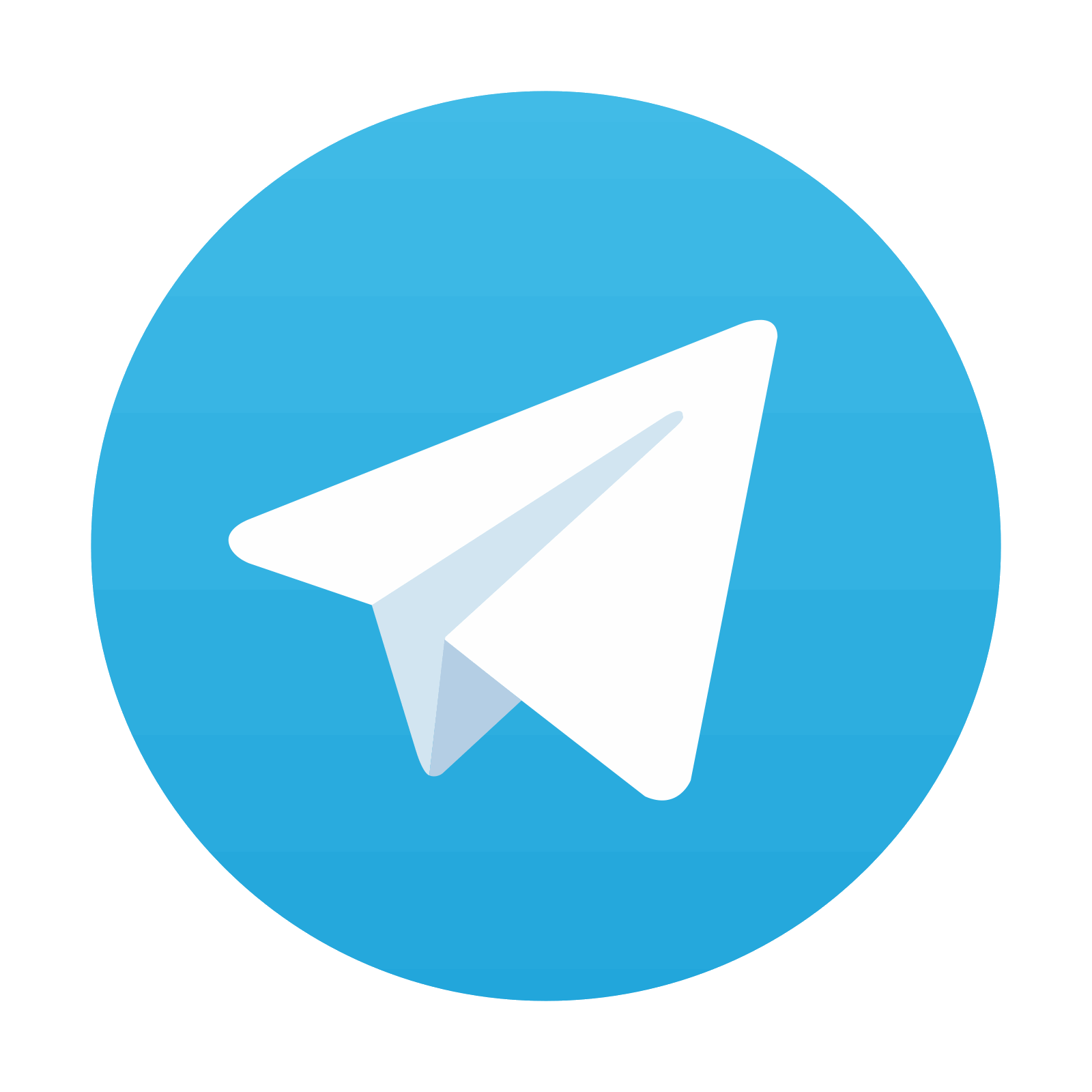
Stay updated, free articles. Join our Telegram channel
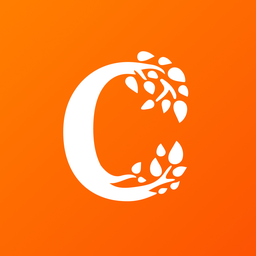
Full access? Get Clinical Tree
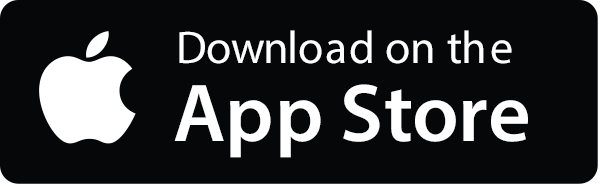
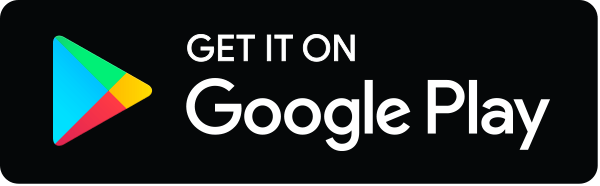
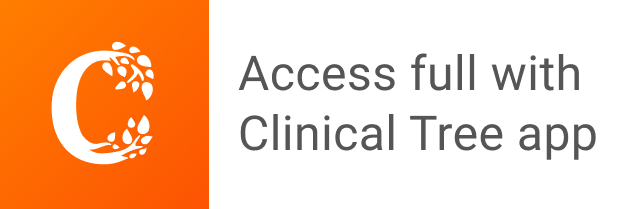