Term
Definition
Biomarker
A characteristic that is objectively measured and evaluated as an indicator of normal biological processes, pathogenic processes, or pharmacologic responses to an intervention
Prognostic biomarker
Intended to forecast a likely disease course in the absence of treatment
Predictive biomarker
Intended to forecast a likely disease course in the presence of a specific treatment
Early response biomarker
Intended to reveal treatment response or failure earlier than conventional methods
Clinical endpoint
A characteristic or variable that reflects how a patient feels, functions, or survives
Surrogate endpoint
A biomarker intended to substitute for a clinical endpoint
Biomarker evaluation
The overall process for confirming a biomarker’s suitability as a surrogate endpoint
Analytical validation
The first component of biomarker evaluation in the IOM framework, describing objective demonstration that the biomarker can be accurately measured
Qualification
The second component of biomarker evaluation in the IOM framework, describing objective demonstration that the biomarker is associated with the clinical endpoint of concern
Utilization
The third component of biomarker evaluation in the IOM framework, describing subjective assessment of biomarker performance in the specific context of its proposed use
Any discussion of biomarkers in the context of oncology clinical trials must also include definitions of clinical and surrogate endpoints. The NIH Biomarkers Working Group defines a clinical endpoint as “a characteristic or variable that reflects how a patient feels, functions, or survives” [4]. Within a clinical trial, a clinical endpoint is a distinct measurement or analysis of disease characteristics that reflects the effect of a therapeutic intervention. In general, survival has traditionally been the clinical endpoint of greatest interest in oncology trials [5].
A surrogate endpoint is defined as a biomarker that is intended to substitute for a clinical endpoint [4]. Surrogate endpoints are a subset of biomarkers. Using a biomarker as a surrogate endpoint implies that the biomarker has been through a rigorous, formal process to confirm its suitability as a substitute for a clinical endpoint. This confirmatory process has been described using a variety of different terms, including evaluation, qualification, and validation. In keeping with the IOM framework, we use the general term biomarker evaluation to refer to the overall process for confirming a biomarker’s suitability as a surrogate endpoint. The IOM framework identifies three key components in the biomarker evaluation process: analytical validation, qualification, and utilization [2]. These three steps are discussed in more detail in Sect. 2.7.
2.3 Motivations Underlying Biomarker Integration into Oncology Drug Development
Despite its relatively recent emergence onto the research agenda, the biomarker concept is now invoked at all stages of oncology drug development, from early preclinical studies to late-stage clinical trials. The search for meaningful oncology biomarkers reflects both the growing importance of precision medicine and the increasing emphasis on finding ways to streamline the drug development process [6].
In the context of oncology drug development, precision (or personalized) medicine captures the notion of cancer as a heterogeneous group of diseases characterized by a diverse array of gene expression and activity patterns [7]. Tumor analysis at the molecular level offers the potential for identifying targets that may be variably expressed among different patients or even at different tumor sites in a single patient. Linking different cancer subtypes with the presence or absence of certain biomarkers may facilitate identification of tumors in which a targeted drug agent has a higher likelihood of success. Biomarker integration may thus promote optimization of therapeutic regimens for individual patients, with specific agents being selected only in tumor subtypes associated with a particular biomarker status, e.g., trastuzumab for HER2-overexpressing breast cancers or cetuximab for EGFR-expressing colorectal cancers lacking a concomitant KRAS mutation.
Meanwhile, integration of biomarkers into preclinical studies and clinical trials offers the potential of reducing the length and expense of the drug development process, which by some estimates can require up to 10 years and one billion dollars [8]. In preclinical and early-stage clinical development, biomarker analysis may promote improved selection of patients for clinical trials by identifying patients who are more or less likely to benefit from therapy. Biomarkers may provide valuable early information on the presence or absence of drug efficacy that can be used to terminate less promising projects before they enter into more expensive later-stage testing [6]. Performing such studies in the preclinical setting also affords the opportunity to select appropriate imaging biomarkers for application in subsequent clinical trials employing the therapy under consideration.
For investigational agents that do proceed into late-stage clinical testing, biomarkers used as surrogate endpoints offer the prospect of smaller, less expensive, and more efficient clinical trials [9]. In particular, if a biomarker is deemed to be an acceptable substitute for survival, then trials can be designed and powered to demonstrate a significant change in the biomarker rather than the clinical endpoint, typically resulting in smaller patient accrual requirements and a dramatically shortened evaluation timeframe. Furthermore, traditional survival trials in many cancer types are becoming more difficult to analyze and interpret due to increasing patient life expectancies and the proliferation of therapeutic options, e.g., in metastatic breast cancer where patients may undergo multiple different lines of treatment extending over several years. It has been argued that in certain circumstances, biomarker-driven trials may offer “cleaner” assessments of drug efficacy than survival trials with fewer problems due to patient loss to follow-up, patients undergoing additional treatment after the investigational therapy, and patients experiencing intercurrent illness and death from other causes [9, 10].
2.4 Use of Imaging Biomarkers Across the Oncology Drug Development Continuum
An important concept to emphasize is that different imaging biomarkers might be appropriately deployed at different stages of the oncology drug development process. As the goals change from preclinical studies into early- and late-stage clinical trials, the requirements for biomarker evaluation and utilization also change.
2.4.1 Preclinical Studies
In the preclinical stages of the drug development process, key priorities include target identification and validation, identification of promising drug compound leads, and demonstration of target engagement as proof of concept. This process typically occurs first in in vitro studies of appropriate cell lines and then in preclinical in vivo animal studies and may initially involve high-throughput screening of thousands of candidate compounds to identify a handful of promising leads. The most sought-after imaging biomarkers during the preclinical stage will provide information on activity at the cellular and molecular levels. A comprehensive review of imaging biomarkers for preclinical drug development is beyond the scope of this chapter, which is focused on imaging biomarkers for human clinical trials.
2.4.2 Early- and Late-Stage Clinical Trials
Investigational agents undergo increasingly rigorous clinical testing as they progress along the path toward regulatory approval. Phase 1 and 2 studies are typically smaller trials designed to establish initial safety and dosing data and to demonstrate efficacy in small study populations. Phase 3 studies are typically much larger and more costly multisite trials aimed at collecting the necessary safety and efficacy data to support a marketing application to regulatory authorities. Given the expense involved in proceeding from phase 2 into phase 3 testing, a key objective in early clinical trials is gathering sufficient preliminary efficacy data to inform a “go or no-go” decision on continuing into phase 3 studies.
The most relevant imaging biomarkers during clinical trials will be those that provide evidence of drug efficacy as well as those that select for patient subgroups in whom drug efficacy may be higher [11, 12]. In phase 1 and phase 2 trials, investigators may choose to incorporate biomarker endpoints from a constantly expanding menu of advanced imaging techniques, presumably selecting approaches that report on functional or compositional variables correlated with the mechanism of drug action. It is in this context that the advanced techniques described in this text are currently most relevant for demonstrating the efficacy of novel molecularly targeted agents. In phase 3 trials, the most important biomarkers will be those that are reproducible across large, multisite trials and those that have been rigorously confirmed as acceptable surrogate endpoints for survival. The requirements for imaging biomarkers may be more stringent for late-stage than for early-stage clinical trials because biomarker results from early-stage clinical trials are used primarily by the trial sponsor for internal decision making, while results from late-stage clinical trials will be scrutinized by outside regulatory authorities in the drug approval process.
2.5 Current Imaging Biomarkers for Oncology Clinical Trials
Imaging biomarkers for oncology clinical trials have evolved over the past 50 years, driven by the need for objective standards with which to perform “apples-to-apples” comparisons of treatment response both between patients within a clinical trial and between different clinical trials. Until recently, the majority of imaging biomarkers for oncology have centered on tumor size measurement and size measurement changes. This section briefly reviews the salient features and drawbacks of size-based imaging biomarkers, with an emphasis on the Response Evaluation Criteria for Solid Tumors (RECIST), the most commonly recognized and utilized standard for assessing response in solid malignancies. We also review the important criticisms of RECIST, and we describe incremental modifications of RECIST that have been deployed in various tumor types.
2.5.1 RECIST
Imaging-based tumor size measurement assessment guidelines for solid malignancies were first codified in the 1980 World Health Organization criteria [13] and were revised as the Response Evaluation Criteria in Solid Tumors (RECIST) in 2000 [14] and RECIST 1.1 in 2009 [15]. The Macdonald criteria for supratentorial malignant glioma were proposed in 1990 [16]. The International Working Group (IWG) or Cheson criteria for hematologic malignancies were first issued in 1999 [17] and were revised in 2007 [18]. These response assessment tools have enjoyed widespread utilization in the scientific, industrial, and regulatory communities, and new drug approval applications routinely include results using these imaging biomarkers to support efficacy claims.
RECIST was explicitly designed for use in phase 2 clinical trials (although it is used at other stages in drug development and even clinically) and is essentially a guideline for assessing tumor response and progression based on changes in anatomical tumor burden over time. RECIST specifies criteria for categorizing lesions on baseline (i.e., pretreatment) imaging as either “target” or “nontarget” lesions, with the former to be followed with successive quantitative size measurements and the latter to be followed qualitatively. At follow-up imaging (i.e., during treatment), lesion burden is reassessed in standardized fashion, with target lesions reevaluated as the sum of their unidimensional size measurements and nontarget lesions reevaluated subjectively according to changes perceived by the reviewer. At each imaging timepoint (typically a predetermined follow-up interval specified in the study protocol), patients are assigned one of four response categories: complete response (CR), partial response (PR), stable disease (SD), or progressive disease (PD). These patient-level response categories can then be used to construct summary trial endpoints including objective response rate (ORR, i.e., the percentage of patients achieving either PR or CR), time to progression (TTP, i.e., average time until PD), and progression-free survival (PFS, i.e., average time until PD or death).
As present time, most clinical trial protocols for solid malignancies specify one or more of these RECIST-derived imaging biomarkers as endpoints for assessing efficacy of the investigational agent. Although ORR is still widely utilized, PFS and other “time-to-event” biomarkers are increasingly incorporated as primary efficacy endpoints, especially in late-stage randomized clinical trials [19]. Recent National Cancer Institute (NCI) task force recommendations have specifically encouraged the use of PFS as a primary efficacy endpoint in phase 2 clinical trials [20]. The ascendancy of PFS has paralleled the introduction of cytostatic agents into the therapeutic armamentarium; these agents, in contrast to traditional cytotoxic agents, result in cell cycle arrest rather than cell death and may be less likely to produce gross tumor shrinkage, although patients may benefit from a delay in tumor progression which would be captured in PFS but not in ORR.
2.5.2 Problems with Size-Based Biomarkers
How well do tumor size-based biomarkers perform for response assessment? In the phase 2 clinical trial setting, ORR is generally accepted as a valid indicator of antitumor efficacy because objective responses are infrequent in the absence of efficacious treatment [21]. There also exists a small but important literature linking objective tumor response to clinical survival benefit [22, 23], to success in later-stage clinical testing [24], and to future regulatory approval [25]. In general, however, tumor shrinkage is considered an unreliable surrogate for survival, one that may either overestimate or underestimate a drug’s effect on the relevant clinical endpoint [15, 26] and one that may have a different correlation with survival in different tumor types or with different drug agents [25, 27]. PFS, meanwhile, has been correlated with survival only in certain tumor types (particular advanced colorectal and ovarian cancers) [28], and biostatisticians have cautioned against extrapolating survival associations even in these tumor types to novel anticancer therapies [27]. Although tumor size-based endpoints have become an important basis for oncology drug regulatory approval [21, 29], there have been several high-profile examples of authorities having granted accelerated approval to new anticancer drugs based on tumor size measurement data, only to rescind or narrow the approval as postmarketing data failed to show a survival benefit; notable examples include U.S. Food and Drug Administration (FDA) approvals of gefitinib (Iressa) for non-small cell lung cancer and, more recently, bevacizumab (Avastin) for metastatic breast cancer.
The shortcomings of RECIST have been well publicized [30, 31] and fall into two general categories: practical problems with its implementation and more fundamental objections to a size-based approach to response assessment. Practical problems with implementing RECIST include difficulties choosing target lesions representative of total tumor burden, the need to assess many lesions on a qualitative and subjective basis, and high intraobserver and interobserver variability for heterogeneous lesions or lesions with irregular borders [32]. Single-axis measurements as dictated by RECIST may not adequately capture size changes in nonspherical lesions or lesions with asymmetric growth. Lesions along curved surfaces, abutting other organs, or adjacent to other pathology may be difficult to measure by RECIST guidelines. Finally, the use of categorical rather than continuous response variables may sacrifice statistical power.
A more fundamental objection to RECIST is that an exclusive focus on tumor size may exclude other potentially meaningful features, including morphologic, compositional, and functional parameters that may provide a more comprehensive assessment of tumor status. Tumor size change may lag weeks to months behind a tumor biological response or may never occur at all. Size measurement criteria may therefore underestimate or fail to capture antitumor efficacy, especially of newer targeted agents that produce a cytostatic rather than cytotoxic effect.
These considerations are motivating ongoing efforts within the oncologic imaging community to improve upon current tumor size measurement biomarkers. These efforts fall into two general categories: incremental modifications to size-based biomarkers and novel imaging techniques reporting on parameters other than tumor size.
2.5.3 Incremental Modifications to Tumor Size Measurement Techniques
Other important modifications of tumor size measurement techniques under current investigation include three-dimensional volumetric measurement approaches [33] and customized response assessment guidelines tailored to specific tumor types. Examples of the latter include the Choi criteria for gastrointestinal stromal tumors [34], the modified RECIST (mRECIST) criteria for hepatocellular carcinoma [35], and the immune-related response criteria (irRC) for melanoma immune modulator therapies [36].
In addition, the RECIST and IWG criteria themselves are dynamic systems that continue to evolve and incorporate new techniques. The most recent IWG criteria incorporate fluorodeoxyglucose positron emission tomography (FDG-PET) on a variable basis depending on the FDG avidity of the lymphoma subtype [18]. RECIST 1.1 also incorporates FDG-PET, albeit on a limited basis as an indicator of disease progression [15]. In general, despite much enthusiasm for the potential of metabolic imaging, response assessment guidelines have been slow to incorporate FDG-PET, especially for solid malignancies. This is due, at least in part, to the great challenge in optimizing and standardizing techniques to enable comparable results to be obtained by different vendors and institutions.
2.6 Emerging Techniques
The cancer imaging community is actively engaged in developing new oncologic imaging biomarkers based on advanced methods of tumor characterization. Development of new biomarker tools has proceeded across several different modalities, but in general all of the newer methods aim to interrogate for functional, molecular, or compositional changes that may report on tumor response earlier and/or with greater specificity than conventional methods. Emerging techniques providing candidate biomarkers include perfusion imaging (including dynamic contrast enhancement-MRI (DCE-MRI), perfusion CT, and microbubble contrast ultrasound techniques), diffusion imaging (including newer whole-body MR diffusion approaches), advanced imaging tools for molecular compositional analysis (including MR spectroscopy, magnetization transfer, and chemical exchange saturation transfer techniques), new elastography approaches (both MR and ultrasound based), and hybrid techniques facilitating registration of functional and anatomical information (including PET-CT and PET-MR). A brief introduction is presented here, but the reader is referred to other chapters in this volume for more detailed information on these techniques.
2.6.1 Imaging Methods Reporting on Vascular Status
When a malignant tumor reaches approximately 1–2 mm3 in volume, it can no longer rely on the passive diffusion of metabolites from host tissue blood vessels in order to continue to proliferate, so new vasculature must develop in order for the tumor to continue to thrive [37, 38]. This process of neovascularization or angiogenesis is a signature of neoplasms and one of the principal potential targets for quantitative imaging [39]. In contrast to mature blood vessels that are the result of normal physiologic processes, tumor vessels produced by angiogenesis are characteristically leaky, fragile, and incompletely formed. It is believed that virtually all solid tumors are dependent upon angiogenesis for survival [40] and many anti-angiogenic drugs are currently in clinical trials [41]. Thus, methods for imaging and quantitatively assessing this phenomenon are quite promising as biomarkers for application in preclinical and clinical studies.
Currently, one of the most widely employed methods for characterizing tumor neovasculature is DCE-MRI. (Other important methods for interrogating tumor vascularity include contrast-enhanced CT [42] and microbubble enhanced sonography [43].) Changes in the parameters obtained from DCE-MRI can be used to assess vascular changes within a tumor and, in particular, how a tumor is responding to treatment. The method is based on measurements and pharmacokinetic models of how a (typically) gadolinium based contrast agent perfuses through such vessels. Healthy vessels in normal tissues may be characterized by a range of parameters measuring blood flow, vessel permeability, and tissue volume fractions (i.e., fractions of a given sample of tissue that can be attributed to intravascular or extravascular space). These parameters are known to be different in vessels associated with tumors. Furthermore, as tumor blood vessels are known to change in response to anti-angiogenic drugs, the method provides a way of quantifying those changes. It is thus a plausible hypothesis that parameters measuring treatment induced changes in pathologic vessels will be predictive of response at an earlier time than changes in longest dimension. Indeed, many studies across a range of tumor types have shown just that.
2.6.2 Imaging Methods Reporting on Cell Density
Perhaps the most basic definition of cancer is that it is a set of diseases characterized by unregulated cell growth and proliferation. Furthermore, since many anticancer drugs have as their ultimate goal destruction of tumor cells, imaging methods sensitive to changes to tissue cellularity are of great importance. The typical application of histology and molecular biology in living systems is somewhat limited as it cannot provide a noninvasive deep tissue visualization of cells and molecules of interest. In particular, the visualization of cellular and molecular activity in animals normally requires the sacrifice and destruction of the organism to allow for analysis by histology and molecular biology. The development of cellular and molecular imaging techniques has begun to bridge this gap.
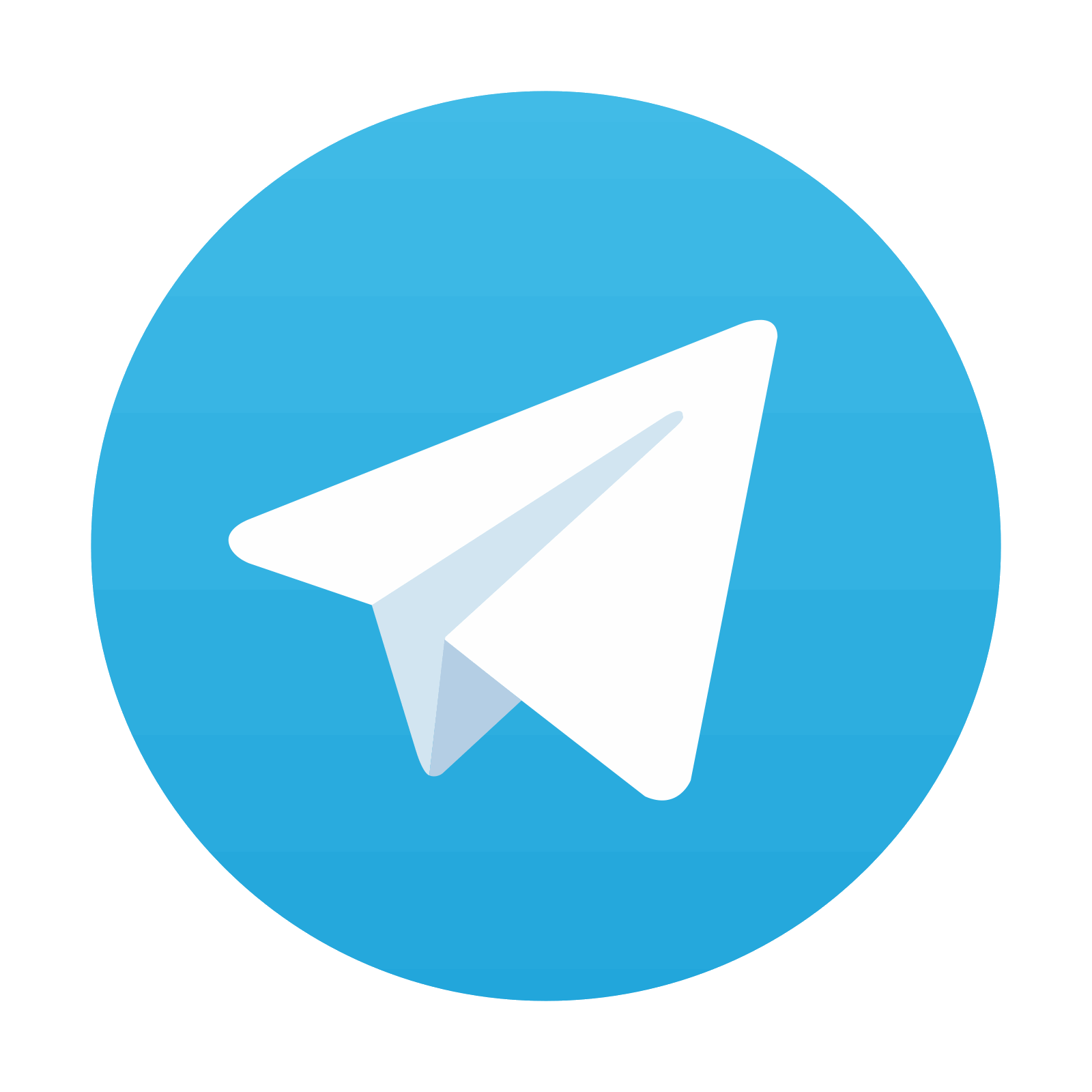
Stay updated, free articles. Join our Telegram channel
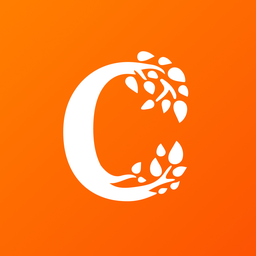
Full access? Get Clinical Tree
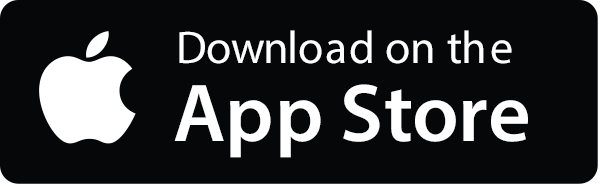
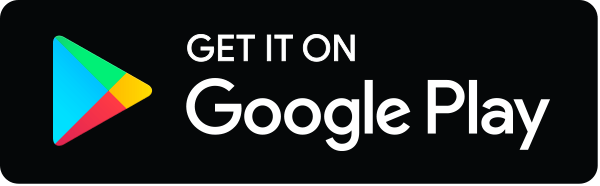