Fig. 1
Approximate time course of clinical manifestations following exposure to levels from 1 to over 2 Gy. George et al. (2007)
The early or prodromal phase response from exposure to ionizing radiation is characterized by a dose-dependent expression of a constellation of signs and symptoms including nausea, vomiting, anorexia, and central nervous system function impairment. An early prodromal phase developed during the first week is followed by a clinically silent phase, which is shorter after higher doses. The appearance of acute symptoms, such as vomiting, is directly dependent on the radiation dose to an overexposed individual and contributes to the diagnostic index used for assessing dose as shown in Table 1.
Dose Estimate | Time to Onset of vomiting | |
---|---|---|
Gy | % | Time(hr) |
0 | – | – |
1 | 19 | – |
2 | 35 | 4.63 |
3 | 54 | 2.62 |
4 | 72 | 1.74 |
5 | 86 | 1.27 |
6 | 94 | 0.99 |
7 | 98 | 0.79 |
8 | 99 | 0.66 |
9 | 100 | 0.56 |
10 | 100 | 0.48 |
Reddening of the skin, or initial erythema, is generally seen within a few hours to a few days following exposure to a high radiation dose (>2 Gy) and lasts only for up to 48 h. When caring for such a patient the location and time course of radiation-induced cutaneous injury should be recorded. This information provides diagnostic information concerning partial- or whole-body exposures and can later help to define the boundary of skin grafts. The manifest illness phase becomes clinically evident as a consequence of the impairment of some critical organ systems such as neurovascular(N), hematopoietic(H), cutaneous(C), and gastro-intestinal(G).
The hematopoietic syndrome consists of alternation in hematopoiesis due to damage of stem cells in marrow and lymphatic tissue. The changes may vary from mild to lethal. A decrease in lymphocytes occurs promptly, mostly taking place within 24 h after exposure. The level of this early lymphopenia is one of the best indicators of the severity of radiation injury. The level of neutrophil granulocytes shows a very early rise, usually limited to the first 48 h or less. In the dose range that produces the hematopoietic syndrome, after the early rise, granulocyte numbers fall to fairly low levels at about day 10, and there is then transient, abortive rise at around day 15, perhaps due to mitoses of a genetically damaged population that cannot continue to reproduce.
Table 2 summarizes the clinical response of these syndromes. After exposure to ionizing radiation these four early reacting organ systems express different signs and symptoms. After very high doses, the prognoses of ARS depend mainly on the extent of damage to organs other than bone marrow (e.g., lung, gastrointestinal tract and skin), with the risk of multiple organ dysfunction (MOD) and even multiple organ failure (MOF).
Table 2
Grading system for response of neurovascular, gastrointestinal, cutaneous, and hematopoietic systems (Jamle et al. 2004)
Symptom Neurovascular system | Degree 1 | Degree2 | Degree3 | Degree4 |
---|---|---|---|---|
Nausea | Mild | Moderate | Intense | Excruciating |
Vomiting (per day) | Occasional (once) | Intermittent (2–5 times) | Persistent (6–10 times) | Refractory (>10 times) |
Temperature, °C | <38 | 38–40 | >40 for <24 h | >40 for >24 h |
Headache | Minimal | Moderate | Intense | Excruciating |
Blood pressure(mm Hg) | >100/170 | <100170 | <90/60; transient | <80/?: persistent |
Neurologic deficits | Barely detectable | Easily detectable | Prominent | Life-threatening, loss of consciousness |
Cognitive deficits | Minor loss | Moderate loss | Major impairment | Complete impairment |
Gastrointestinal system Diarrhea | ||||
Frequency, stools/d | 2–3 | 4–6 | 7–9 | ≥10 |
Consistency | Bulky | Loose | Loose | Watery |
Bleeding | Occult | Intermittent | Persistent | Persistent with large amount |
Abdominal cramps or pain | Minimal | Moderate | Intense | Excruciating |
Cutaneous system | ||||
Erythema | Minimal, transient | Moderate (<10 % body surface area) | Marked (10–40 % body surface area) | Severe (>40 % body surface area) |
Sensation or itching | Pruritus | Slight and intermittent pain | Moderate and persistent pain | Severe and persistent pain |
Swelling or edema | Present, asymptomatic | Symptomatic, tension | Secondary dysfunction | Total dysfun ction |
Blistering | Rare, sterile fluid | Rare, Hemorrhage | Bullae, sterile fluid | Bullae, hemorrhage |
Desquamation | Absent | Patchy dry | Patchy moist | Confluent moist |
Ulcer or necrosis | Epidermal only | Dermal | Subcutaneous | Muscle or bone involvement |
Hair loss | Thinning, not striking | Patchy, visible | Complete, reversible | Complete, Irreversible |
Onycholysis | Absent | Partial | Partial | Complete |
Hematopoietic system | ||||
Lymphocyte changes (cells/L) | ≥1.5 × 109 | 1–1.5 × 109 | 0.5–1 × 109 | <0.5 × 109 |
Granulocyte changes (cells/L) | ≥2 × 109 | 1–2 × 109 | 0.5–1 × 109 | <0.5 × 109 |
Thrombocyte changes (cells/L) | ≥100 × 109 | 50–100 × 109 | 20–50 × 109 | <20 × 109 |
2.2 Mathematical Dose Reconstruction
Mathematical dose reconstruction using Monte Carlo analysis is a scientifically acceptable means of performing a retrograde or retrospective analysis of the previous dose or exposure of an individual (Takahashi and Endo 2007). If the source type, activity, position (external), incorporation path (internal), and exposure time can be estimated, then detailed calculations of the dose can be made. Above all, calculations based on radiation transport code are becoming one of the most significant tools in the field of radiation protection dosimetry because of progresses in technologies concerning computation. In addition, a technique using a radiation transport code has the advantage of providing an estimation of radiation doses at any point inside the human body. Therefore, it is used in a wide range of application such as therapy, diagnosis and protection.
Monte Carlo analysis is often thought of as computer “experiment”. The method employs random numbers to select probabilities of particles interactions along with resultant energies and directions of particle flight after collisions. Monte Carlo codes are usually written for three-dimensional geometries and capable of supporting complex geometrical definitions. Using this type of code, all elements of geometry can be fully described in three dimensions, and the simulation is effectively acting similar to an experiment. There are a number of computer codes available, for example MCNP, MCNPX, SABRINA, EGS, and ITS, etc., that have been used for radiation accident reconstruction.
Selection of an appropriate model is essential if numerical analysis is used for the accurate estimation of the radiation doses to exposed persons following an accident. Several complicated procedures and assumptions are required to prepare an input file, defining the model in the radiation transport codes. Monte Carlo calculation methods are used to determine the energy deposited in different “tissues” of the mathematical phantom by following millions of particles (betas, photons, neutrons) from their points of origins to their points of absorption or transmission.
Two primary phantom-based methodologies are available for representing human body tissues in Monte Carlo calculations. These are voxelized phantoms, which are based on human CT or MR images of organs and tissues, and mathematical models where body counters and organs are defined by mathematical expressions, as shown Fig. 2. Most of the computational models of the human body commonly in use are the so-called mathematical models: mathematical expressions representing planes, cylindrical, conical, elliptical, or spherical surfaces are used to describe idealized arrangements of body organs. The paradigm of the mathematical models is commonly called the “MIRD-5 phantom”, due to being published in the MIRD Pamphlet No.5. Referring to this, mathematical models are often called MIRD-type models. Recently, tomographic models have been developed, which use computed tomographic (CT) or magnetic resonance (MR) data of real human subjects to provide three-dimensional representation of the body. Each organ or tissue is represented by those volume elements (voxels) which were identified as belonging to it from the CT or MR slice images. This tomographic type of model is called a voxel (volume pixel) model.
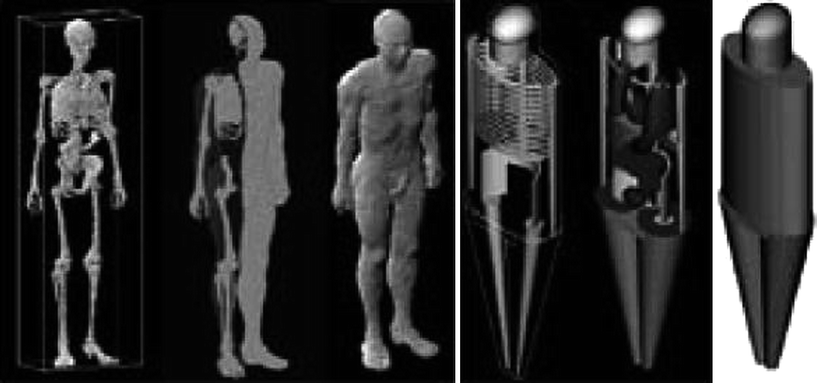
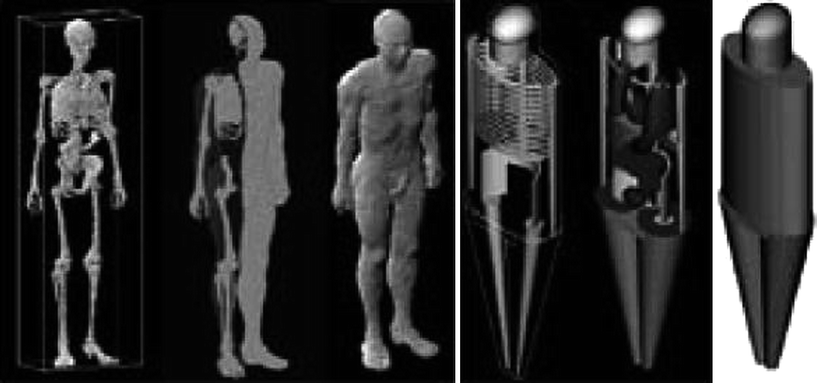
Fig. 2
Comparison between the NORMAN voxel phantom and ADAM-MIRD mathematical model (Ferrari and Gualdrini 2005)
In mathematical models, organ shapes are reduced to a very simple form to limit the software and computational requirements. Consequently, the mathematical models are not designed to describe any individual in detail but rather to represent whole populations. On the other hand, tomographic models are constructed from CT or MR data of real persons who might deviate significantly from reference data. The shapes of the body organs are determined by identifying all the voxels belonging to each organ. Thus, the shape of each organ is more realistic than for the mathematical models, although, being reconstructed from a specific individual, it might not be representative of large populations.
Radiation paths in the anthropomorphic phantoms are usually calculated using Monte Carlo codes following individual particle histories. For each single particle history, the parameters influencing its actual course are selected randomly from their probability distributions. There are libraries of cross-section data for the radiation interaction processes for all elements from which the cross-section data for body tissues are then evaluated according to their elemental composition and density. Organ absorbed doses are evaluated by summing, in each organ and tissue, all energy depositions from primary and secondary particles and dividing by the organ mass. This results in the average absorbed dose in the organ regardless of the irradiated fraction.
2.3 Retrospective Dosimetry
Retrospective dosimetry may be estimated from materials and objects carried or in close proximity to the exposed individual. The dosimetric techniques include activation analysis, thermoluminescence, optically stimulated luminescence, and electron spin (or paramagnetic) resonance. Unless the absorbing material was in very close proximity to the victim, dose reconstruction will still be required to relate the dose received by any particular material to that received by the victim.
The common objects assessed by activation analysis following a criticality accident are metal objects worn or carried by the victim that become activated by neutron capture. Any personal metal object on the victim can be used, for example rings, keys, coins, a cellular telephone, pager, or a camera, etc. Activation analysis may also be performed in vivo. Neutron capture by stable Na-23 in the body produces Na-24, a beta and gamma emitter with a physical half-life of 15 h and gamma-ray energies of 1.37 and 2.75 MeV. The activity of Na-24 in the victim’s body may be directly assessed by whole-body counting, or the activity in a blood sample may be assessed by gamma-ray spectrometry.
Luminescent materials are crystalline and able to store part of the energy imparted to the material by interaction with ionizing radiation. When ionizing radiation interacts with an insulation crystal, a redistribution of charge within the crystal takes place. Some part of the redistributed charge becomes trapped at defects in the crystal lattice. This redistribution of charge continues for the duration of the radiation exposure and the amount of trapped charge is related to the total radiation exposure. The trapped charge may be released when the crystal is exposed to either heat or light. A fraction of the released charges will recombine, which results in the emission of a tiny light flux called luminescence. The intensity of the emitted luminescence is related to the amount of trapped charge and hence to absorbed dose. If heating is applied to release the trapped charges, the luminescence is called thermoluminescence (TL); if light exposure is the releasing agent the light emission is called optically stimulated luminescence (OSL).
Thermoluminescence is the physical phenomenon in which a solid sample absorbs energy while irradiated at a given temperature, and release this energy in the form of light while heating the sample. The emitted light is recorded as intensity versus temperature in the shape of one or more TL peaks. Under favorable conditions, the emitted TL light intensity is proportional to the absorbed dose, and thus, using an appropriated calibration, one can evaluate the applied dose in the given radiation field.
The sensitivity of a thermoluminescence dosimeter (TLD) depends upon the type of TLD used and the orientation of the TLD with respect to the source of radiation. The TLD dose is generally assigned as a whole-body dose, unless the dosimeter is used in a specific fashion, such as a ring dosimeter, which would have its dose recorded as an extremity dose. If a TLD is available after a radiation exposure, this provides an important basic dose measurement, to which other dose estimated will be compared. The TLD is considered a passive, integrating dose-measuring device. For example, this dose reconstruction method was used to estimate doses received by A-bomb survivors in Hiroshima and Nagasaki by the analysis of bricks and tiles taken from buildings. After any radiation accident, or incident samples of such materials may be gathered from the location and subjected to this type of analysis.
More recently, the use of optically stimulated luminescence (OSL) for dosimetry as well as archeological and geological dating has become very popular. The potential of electronic devices for retrospective, accident, and forensic dosimetry has been demonstrated using optically stimulated luminescence (OSL). Electronic devices contain specific components which can be used as dosimeters. For example, resistors with alumina substrate from a cellular phone have been used to illustrate the case for accident and retrospective dosimetry. Although it is important to search for common components in different kinds of devices, it is of equal importance to be able to perform rapid dose assessments using less common components in electronic device that would be available at the accident site.
Exposure of biological tissue to ionizing radiation results in radiation-induced changes that can be measured and, depending on the absorbed dose, quantified. Electron paramagnetic resonance (EPR) has been used to determine doses to accident victims, based on the capability of the technique to specifically and sensitively measure unpaired electron species (IAEA-TEDOC-1331 2002). While the lifetimes of these species are very short in aqueous system such as most biological tissues, the radiation-induced signals can be extremely stable in non-aqueous media, including teeth, bone, fingernails, and hair. In addition, the production of free radicals by ionizing radiation can lead to the disruption of electron pairing in many substances, which include plastics, clothing (cotton and polypropylene), medicinal tablets, and sugar. The resulting paramagnetic absorption intensity is proportional to absorbed dose and can be measured with an EPR spectrometer. EPR was subsequently used for in vitro retrospective analyses of exfoliated teeth for measuring absorbed doses in populations from Japan and the Former Soviet Union.
EPR consists of the resonant absorption of electromagnetic energy at electron-spin levels. Unpaired electrons of free radicals have spin equal to 1/2. In a magnetic field there are two magnetic levels, +1/2 and −1/2 with different energies. The transition between two these levels is possible under following resonance condition.


where ν is resonance frequency, h is Plank’s constant, g is the g-factor, which is a constant approximately equal to 2 for spin of 1/2, μ B is the Bohr magneton, which is the elementary electronic magnetic moment, and, B is the magnetic field induction. An important conclusion derived from this formula is the linear dependence between the applied magnetic field and the resonance frequency. The most frequently used microwave energies lie in what is called the X band. This is because it is a good compromise between sensitivity, sample size, and water content effects. For X band
and
.


The first derivative of the resonance microwave absorption is commonly the EPR signal as shown in Fig. 3. It is characterized by the following parameters: peak-to-peak line width, ∆B; resonance field, B r ; peak-to-peak amplitude, A.
2.4 Biological Dosimetry
Biological dosimetry is a method of measuring the amount of ionizing radiation dose received by individual using biological materials. This type of dosimeter is useful when an individual is accidentally exposed and no physical dosimetry is available, or if the reading from a physical dosimeter is in dispute.
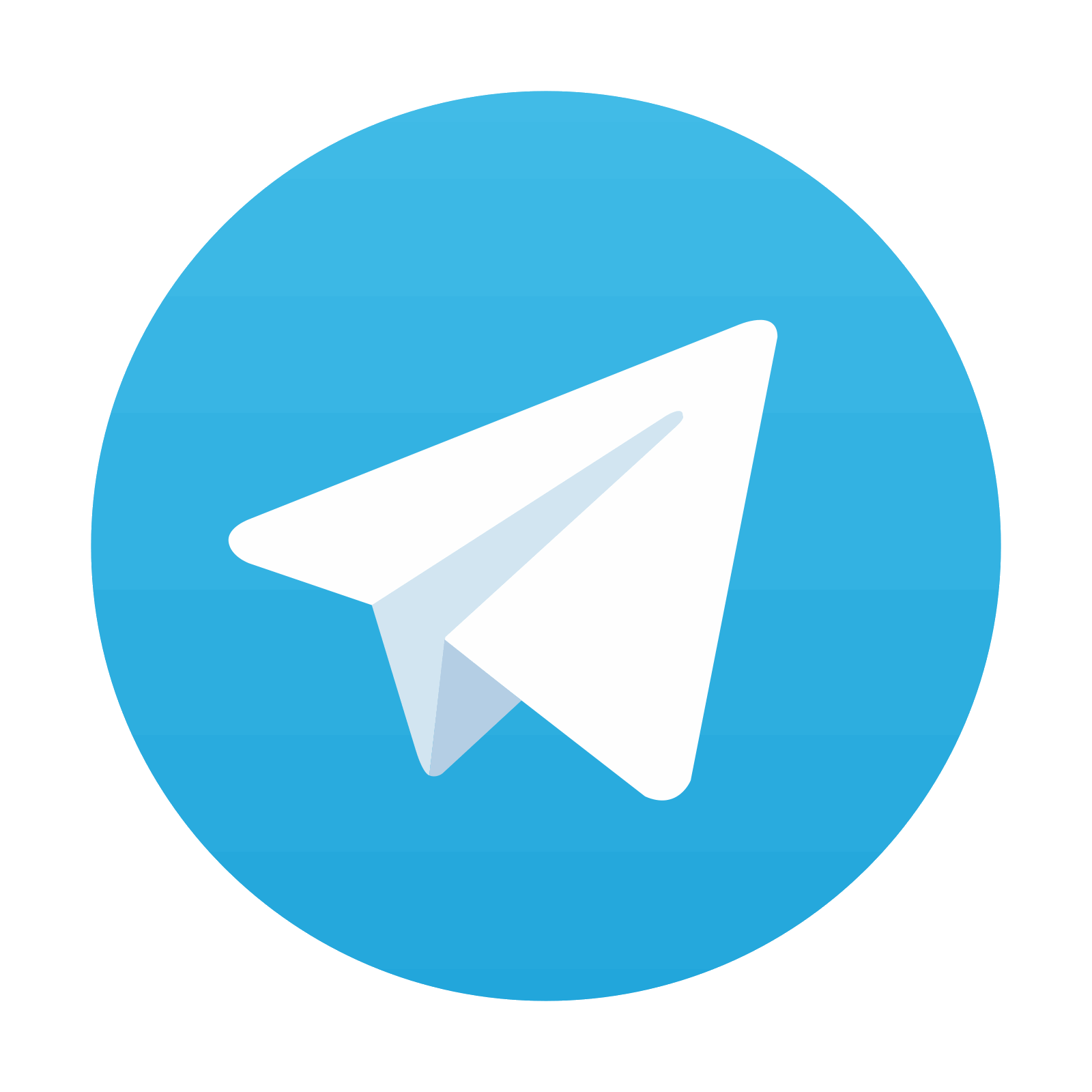
Stay updated, free articles. Join our Telegram channel
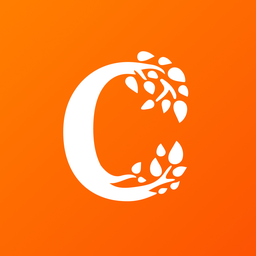
Full access? Get Clinical Tree
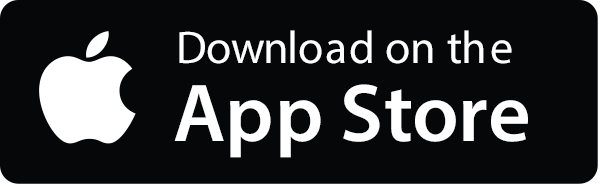
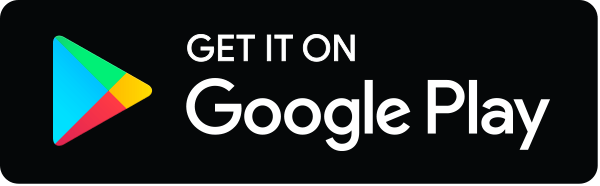