Radionuclide
Emission
Half-life
Range
Approximate cell diameters
131Iodine
ß
8.0 d
0.08–2.3 mm
10–230
90Yttrium
ß
64.1 h
4.0–11.3 mm
400–1100
177Lutetium
ß
6.7 d
0.04–1.8 mm
4–180
188Rhenium
ß
17.0 h
1.9–10.4 mm
200–1000
67Copper
ß
61.9 d
0.05–2.1 mm
5–210
211Astatine
α
7.2 h
60 μm
6
213Bismuth
α
46 min
84 μm
8
125Iodine
Auger
60.5 d
<100 nm
(1)
111Indium
Auger
3.0 d
<100 nm
(1)
3.2 Labeling Strategies
3.2.1 Labeling with Iodine Isotopes
For more than three decades, iodine-labeled antibodies are the most investigated substances for RAIT. The direct electrophilic substitution of antibodies and fragments can be carried out very easily in high yields (Fig. 1).
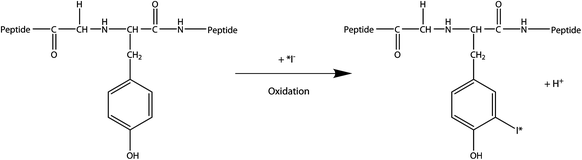
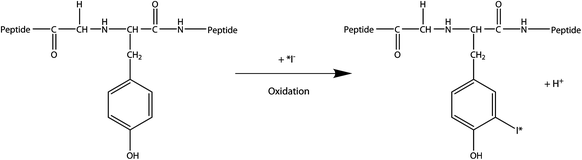
Fig. 1
Radioiodination of tyrosine residuals
In an IgG antibody, normally 4–6 protons of the aromatic peripheral tyrosine or histidine groups are substituted by iodine atoms. Radioiodide isotopes are distributed as alkaline or buffered aqueous solution. Because iodide is relatively inert, most iodinations are carried out by oxidation of iodide to iodonium as intermediate (Bhargava and Acharya 1989).
A number of oxidizing agents have been described for this purpose (Table 2). Today, in most cases, insoluble or immobilised oxidant like Iodogen® are used. These methods allow rapid iodination at the solid phase in aqueous solutions. The incidence of side reactions is negligible or very remote.
Table 2
Examples of iodine oxidant for antibodies labeling
Trade name | Chemical name | Example |
---|---|---|
Iodogen | 1,3,4,6 tetrachloro-3α, 6α-diphenylglycoluril | Delaloye et al. (1997) |
Iodo-beads | N-chloro-benzene sulfonamide coupled covalently to non-porous polystyrene spheres | Stya et al. (1987) |
Chloramin-T | N-monochloro derivative of p-toluene sulfonamide | Stein et al. (2005) |
Based on the covalent binding between the biomolecule and iodide, the labeled products are stable in vitro if the antibody is not internalized into the cells. Large differences in molecular weight between the reacting agents simplify the purification and tend to result in marginal changes in bioreactivity. However, in vivo iodinated antibodies are metabolized to low-molecular-weight molecules which are dehalogenated primarily in the liver. There seem to be several cofactors like circulating antigens or human anti-mouse antibodies (HAMAs) promoting the catabolism (Behr et al. 1996; Geissler et al. 1992). The high emission rate during 131I decay is an additional problem which leads to research for alternative approaches.
3.2.2 Radiometallation of Antibodies
One strategy to overcome the disadvantages of radioiodinated compounds is the complexation of radiometals by antibodies derivatized with multidentate ligands (Table 3). For small tumors, one radiometal of interest is the 67Cu. There are several heterocyclic bifunctional chelators for Cu(II) described which result in thermodynamically and kinetically stable donor–acceptor complexes already at low temperatures. Most of them are 4N macrocycles with either an N-coupled side chain (2-position, Table 2.3, a) or a C-coupled side chain (6-position, Table 2.3, b). The 6-position provides a higher stability in vivo while the precursor synthesis is easier in the 2-position. The reaction between chelators and primary amino groups present at the antibody surface is mediated by reactive groups like iso-thiocyanate or N-hydroxysuccinimide esters which are side-chains-linked at the chelator. In most of the potential chelators additionally contain N-coupled acetate groups which can additionally stabilize the radiometal complex and result in a cage structure (Novak-Hofer and Schubiger 2002).
In a clinical study, the biodistribution of 67Cu-CPTA-mAb35 was directly compared with the 125I-labeled mAb35 in patients with colorectal cancer. This group demonstrated a higher tumor uptake and a higher tumor/blood ratio of the 67Cu-labeled antibody. However, the observed liver uptake was rather high (Delaloye et al. 1997). It has been demonstrated that radiocopper-labeled antibodies are metabolized by proteases and peptidases. The analyses of metabolites resulted in lysine-adducts of the labeled chelator (Rogers et al. 1996). Additionally, the dissociation of Copper from peptides and subsequent binding to superoxide dismutase (SOD) seems to be a problem (Boswell et al. 2009).
Table 3
Examples of bifunctional chelators for radiometallation of antibodies
Structure | Chemical name | Synonym | |
---|---|---|---|
A | ![]() | 4-[(1,4,8,11-tetraazacyclotetradec-1-yl)-methyl]benzoic acid (N hydroxyl-succinimide ester) | CPTA-NHS-ester |
B | ![]() | 2-(4-isothiocyanato-benzyl)-1,4,7,10-tetraazacyclododecane-1,4,7,10-tetraacetic acid | p-SCN-Bn-DOTA |
C | ![]() | N-[(R)-2-Amino-3-(p-isothiocyanato-phenyl) propyl]-trans-(S,S)-cyclohexane-1,2-diamine-N,N,N′,N″,N″-pentaacetic acid | CHX-A″-DTPA |
The analog maximum range of 177Lu and 131I β−-particles in tissue, and its more favorable gamma-ray properties of 177Lu resulted in an intensified interest in this radiometal (Yordanov et al. 2007). Beside DOTA-chelators, the well-investigated open chain DTPA-chelators can be used. DTPA can either be directly coupled to the antibodies or, as already described above, by side-chains-linked reactive groups (Bhargava et al. 1989). 177Lu-DTPA-labeled antibodies were described to be more stable than the DOTA-analogs (Milenic et al. 2002; Yordanov et al. 2007). Almqvist et al. (2006) labeled huA33 with 177Lu using the chelator CHX-A”-DTPA (Table 3, C). Properties of the 177Lu-CHX-A”-huA33 (177Lu-huA33) conjugate was determined both in vitro and in vivo. In nude mice xenografted with colorectal SW1222 tumor cells, a high tumor uptake and high tumor-to-organ ratios could be demonstrated (Almqvist et al. 2006).
3.3 Choice of the Antibody
RAIT is typically given as an intravenous injection, thereby allowing radioactivity to be delivered to tumors throughout the body. Tumor uptake of a radiolabeled monoclonal antibodies (mAb, IgG subtype; ~150 kDa) occurs gradually, taking 1–2 days before peak uptake occurs. Peak uptake is typically <0.01 % of the total injected dose per gram tumor, but the radioactivity deposited in the tumor can be detected several weeks later (Sharkey and Goldenberg 2005, 2006a). Because of its kinetics, the radiation-absorbed dose delivered by RAIT occurs at a much lower dose rate than external beam irradiation, but is continually present for a period of time defined by the physical half-life of the radionuclide and the biological half-life of the antibody residing in the tumor. This continuous, low dose rate radiation exposure can be clinically as effective as intermittent, high dose rate radiation (Hernandez and Knox 2003).
The generation of chimeric and complementarity-determining region (CDR) grafted mAbs have reduced the immunogenicity problem of RAIT with mABs enabling repeated and fractionated therapy. However, humanization prolongs the plasma half time of radiolabeled antibodies, resulting in an increased normal tissue exposure to radioactivity and dose-limiting bone marrow suppression at comparable low activities.
Smaller forms of the antibodies such as F(ab’)2 or Fab’ and more recently, molecularly engineered antibody subfragments with more favorable pharmacokinetic properties are removed more rapidly from the blood, thereby improving tumor/blood ratios (Olafsen et al. 2005; Kenanova et al. 2005). The maximal uptake of smaller antibody constructs may be seen within a few hours after injection, but the affinity of these conjugates is considerably lower than that of a whole antibody (Sharkey and Goldenberg 2006a). As a consequence of their more rapid blood clearance, the fraction of the injected activity delivered to the tumor is lower with an antibody fragment than with a complete mAb of the IgG subtype. Another problem of mAb-fragments is their high retention in the kidneys where they are trapped in even a higher concentration than in malignant tissue (Kenanova et al. 2005).
Complete radionuclide-labeled IgG remains in the blood for an extended period of time, which continually exposes the red marrow to radiation, resulting in dose-limiting myelosuppression. Thus, pretargeting strategies may be used in the future for further dose-escalation studies (Sharkey and Goldenberg 2006a). Unlike antibodies that are directly radiolabeled, pretargeting methods separate the radionuclide from the antibody, thereby allowing time for the antibody to localize in its target before the radionuclide-carrier is given. Two antibody-based pretargeting approaches have been reported, one involving the use of an avidin or streptavidin–biotin targeting system and an approach that uses a bispecific (bs)-mAb (Sharkey and Goldenberg 2005). In each system, the primary targeting mAb carries a secondary recognition moiety, which itself is targeted later with a radiolabeled carrier. The small molecular size of the carrier makes it ideally suited for rapid tumor penetration, and if designed properly, it should clear from the body rapidly (within minutes) without significant residual uptake in non-targeted tissues. First results of a pretargeting approach in the context of RAIT of CEA-expressing tumors in a non-adjuvant setting have already been published (Knox et al. 2000; Forero–Torres et al. 2005; Chong et al. 2005; Kraeber-Bodéré et al. 2006).
3.4 Pathophysiology of Antibody Uptake in Metastatic CRC
In patients with mCRC clinical studies with radiolabeled antibodies without any pretargeting strategy demonstrated, that a tumoricide dose (50–70 Gy) cannot be achieved in larger colorectal cancer metastases (diameter: 10–20 mm). The highest antibody uptake and tumor doses are usually seen in small lesions (Koppe et al. 2005). This clinical observation and dilemma can sufficiently be explained by basic mechanisms of macromolecule transport and physiological pathways.
There are several barriers between the bloodpool and the molecular targets in malignant tissue that have to be passed by radiolabeled antibodies until sufficient therapeutic concentrations of mAbs are present in the tumor. These barriers compass the interstitial subcompartment of a tumor, the walls of the blood vessels and the membranes of cancer and stromal cells. Tumor stroma consists of cells, extracellular matrix (collagen and elastin fiber network) and extracellular molecules. Among the stroma cells, there are fibroblasts, immunocompetent cells, and elements of the vasculature.
Tumor vessels have wide interendothelial junctions, a large number of fenestrae, and transendothelial channels formed by vesicles and discontinuous or absent basement membrane. Lymphatic vessels in a tumor deposit are nearly absent (Jain 1987, 1990, 1994, 2004, 2005; Jain and Baxter 1988). The interstitial pressure of tumors is significantly higher than in normal tissues (Table 4) (Jain 2004) . As the tumor grows, it rises up to >30 mm Hg, presumably due to the proliferation of tumor cells in a confined space and the absence of functioning lymphatic vessels. This increase correlates with a reduction in tumor blood flow and the development of necrosis in a growing (expanding by growth) tumor. Investigations of intratumorale pressure gradients show that the interstitial pressure is higher in the center of a tumor, and it approaches normal physiological pressure toward the periphery.
Table 4
Interstitial fluid pressure (mmHg) in normal and neoplastic tissues in patients after: Jain (2004)
Tissue | Mean | Range |
---|---|---|
Normal skin | 0.4 | −1.0 to 3.0 |
Normal breast | 0.0 | −0.5 to 3.0 |
Head and neck carcinomas | 19.0 | 1.5 to 79.0 |
Lymphomas | 4.5 | 1 to 12.5 |
Lung carcinoma | 19 | −3 to 48.0 |
Colorectal liver metastasis | 21 | 6 to 45 |
Intratumoral blood flow distributions in tumors have been investigated using nuclear magnetic resonance and positron emission tomography. These results show that blood flow rates in necrotic regions of tumors are low, while those in non-necrotic regions are variable and substantially higher than in host normal tissues (Jain 1987, 1990, 1994, 2004, 2005; Jain and Baxter 1988; Netti et al. 2000; Padera et al. 2002, 2004). As a result of these spatial and temporal heterogeneities in blood supply coupled with variations in the vascular morphology at both macroscopic and microscopic levels, the spatial distribution of macromolecules in tumors is heterogeneous and the average uptake decreases with an increase in tumor weight.
Once a macromolecule has extravasated, its movement through the interstitial space occurs mainly by convection. Convection is proportional to the interstitial fluid velocity, which, in turn is proportional to the rate of fluid leakage. Fluid leakage is in turn proportional to the interstitial pressure gradient. Although tumor vessels are “leakier” to fluid and macromolecules compared to several normal tissues, a poor extravasation of macromolecules in tumors is present. Tumors contain regions of high interstitial pressure, which lowers the interstitial pressure gradient and therefore prevent fluid extravasation by convection (Jain 1987, 1990, 1994, 2004, 2005; Jain and Baxter 1988; Netti et al. 2000; Padera et al. 2002, 2004).
While high interstitial pressure prevents convection, diffusion becomes the most important mechanism of extravasation for antibodies. Diffusion is a relatively slow process and mainly proportional to the interstitial concentration gradient. It has been estimated that immunoglobulines transported mainly by diffusion through a tumor deposit will take several days to move for a distance of only 1 mm (Jain 1990).
The movement of an antibody through a tumor deposit is further lowered by the antigen expression of the malignant tissue itself. It is well known that the binding reaction lowers the apparent diffusion rate of molecules. Therefore, although higher affinity of antibody to antigen significantly increases the concentration of the antibody proximal to the vessel, it retards their movement to distal locations in the interstitium (Jain 1990). The effect of antibody degradation within the tumors microenvironment is also of importance.
3.5 The Effect of Anti-CEA Antibodies and RAIT in Tumor Models
Regarding the framework of transport (patho)physiology it is obvious that conventional RAIT using an intact IgG antibody subtype should be certainly more successful in micrometastatic disease than in advanced stages of tumor progression. Additionally, the treatment should be started as early as possible. Apparently, if intact IgG antibodies are able to fulfil their clinical promise, multimodal methods should be developed to overcome the physiological barriers in tumors. These theoretical considerations are also supported from the evidence of preclinical studies (Sharkey et al. 1991; Blumenthal et al. 1992, 2005; Fidarova et al. 2008).
Fiderova et al. used a fluorescently labeled anti-CEA antibody (A5B7) to investigate the kinetics and microdistribution of the antibody in an orthotopic CRC model (LS174T) using high-resolution digital microscopy. The labeled mAb showed rapid, selective uptake into tumor deposits, with a strong negative correlation with tumor size during the first hour post-injection. By 24 h, this correlation was no longer significant because sufficient time had elapsed for equilibration of mAb uptake throughout the majority of larger tumors. The antibody traveled at the same diffusion rate across both small and large tumors, and therefore penetrated to the center of the smaller tumors more rapidly (Fidarova et al. 2008).
Sharkey et al. demonstrated that athymic nude mice receiving intravenous injection of human colonic cancer cells (GW-39) developed multiple (10–100) tumor nodules throughout the lungs. More than 50 % of the animals died of extensive tumor involvement within 5–10 weeks. This could be prevented in most of the animals by the early application of a 131I-labeled anti-CEA antibody NP-4 (Sharkey et al. 1991).
In another study by Blumenthal et al. in the same micrometastatic model of the human colonic carcinoma, lung tumor nodule size, even on the microscopic level, affected therapeutic outcome: radiolabeled anti-CEA antibodies were more effective when administered 7 days post-implantation (mean nodule diameter, 150 μ) compared with the treatment 14 days post-implantation (mean nodule diameter, 750 μ) (Blumenthal et al. 1992).
The same authors used human colon cancer cell lines treated with the anti-CEA mAb IgG1, hMN-14 (labetuzumab). They evaluated direct effects on the proliferation of tumor cells, as well as antibody-dependent cellular cytotoxicity (ADCC) and complement-dependent cytotoxicity (CDC). In vitro, no effect of labetuzumab on apoptosis or tumor cell proliferation directly or by CDC could be observed. In contrast, labetuzumab inhibited the tumor cell proliferation by ADCC (Blumenthal et al. 2005). In vivo studies in nude mice bearing s.c. (local growth) or i.v. (metastatic model) human colon cancer grafts, showed that labetuzumab given as a single agent did not increase the median survival. In contrast, an increased median survival of the mice was observed if labetutzumab was combined with IFN-gamma to up-regulate CEA-expression. Combined therapy of labetuzumab with CPT-11 or 5FU increased the median survival significantly as compared to the drug or antibody alone (Blumenthal et al. 2005).
De Jong et al. (2009) investigated the efficacy of RAIT in the treatment of CRC-LM in an experimental rat model. For therapy 177Lu- and 111In-radiolabeled mAb MG1 (a murine IgG2a antibody, directed against a syngenic colon carcinoma cell line CC531) was used. Administration of 177Lu-MG1 RAIT immediately after surgery improved survival significantly (p = 0.009) compared with the controls, whereas delayed treatment did not (p = 0.94).
4 Radioimmunotherapy in Metastatic CRC: Clinical Studies
To date, more than 20 reports about RAIT in mCRC have been published. Different antibodies (in most trials antibodies against CEA) labeled with either 125I, 131I, 90Y, 177Lu, or 188Re were used. Earlier studies used murine immunoconjugates. During the last 15 years, chimeric and humanized immunoconstructs with lower immunogenity and the possibility of repeated application were developed and are now preferably used. Very recently, pretargeting strategies have entered the clinical practice after the proof of principle in animal studies (Goldenberg et al. 2007; Goldenberg and Sharkey 2008).
Most of the patients in clinical studies were heavily pretreated with chemotherapy ± radiotherapy in advanced disease stages. All were phase I/II trials, single-arm and non-controlled studies, in most cases designed to explore the toxicity of RAIT and to determine the MTD of the radioimmunconjugate. Therapeutic efficacy, however, was also assessed. In the majority of the studies, a single dose regimen of RAIT was used, but fractionated RAIT and repeated RAIT combined with chemotherapy was also carried out, especially if chimeric and humanized mAbs were available (Koppe et al. 2005).
In summary, the clinical studies showed that:
1.
RAIT in CRC-patients is feasible and safe,
2.
Small volume disease shows better response than bulky tumors and has been recognized as the most suitable setting for the application of radiolabeled mabs in CRC-patients,
3.
RAIT in advanced stages of CRC shows only marginal effects on the patients’ outcome,
4.
Further dose escalation beyond a “single shot” therapy can be carried out either by fractionation or by pretargeting strategies.
5.
The value of RAIT in the context of multimodal therapies needs further evaluation.
4.1 Clinical Studies with Radiolabeled Antibodies in Metastatic CRC
4.1.1 Chimeric T84.66 Anti-CEA Monoclonal Antibody (cT84.66 mAb) in mCRC
Chimeric T84.66 (cT84.66) is a high affinity (1.16 × 1011 M−1) and highly specific anti-CEA mAb evaluated over the last years in patients with CRC at a single institution (Neumair et al. 1990). Initial evaluation of biodistribution, pharmacokinetics, toxicities, immunogenicity, tumor targeting, and organ and tumor-absorbed dose estimates of 90Y-cT84.66 during a phase-I study were first reported by Wong et al. (2000). A number of 22 patients (CRC n = 18) with CEA-expressing tumors were treated after dosimetric evaluation with 111In-cT84.66. Dose levels ranged from 5 to 22 mCi/m2. Hematotoxicity was the only dose-limiting side effect of the therapy. The MTD in this study was reached at 22 mCi/m2. Tumor dose estimates ranged from 8.7 to 52.2 cGy/mCi 90Y. Antitumor effects were observed in 5/22 patients. HACAs (human anti-chimeric antibodies) were detected in 10/22 patients (Wong et al. 2000).
The same group used 90Y-cT84.66 in combination with systemic 5FU-based chemotherapy in 21 patients with chemotherapy-refractory mCRC in a phase-I trial. 16.6 mCi/m2 90Y-cT84.66 (single shot) were combined with a 5-day continuous infusion of 5-FU initiated 4 h before antibody infusion (Wong et al. 2003). Dose levels of 5-FU ranged between 700 and 1,000 mg/m2/day. The MTD of the combined therapy was reached at activities of 16.6 mCi/m2 90Y-cT84.66 combined with 1,000 mg/m2/day 5-FU. A number of 11 patients with previously progressive disease stabilized after the treatment for 3–8 months. In addition, concomitant chemotherapy seemed to reduce the incidence of HACAs compared to patients treated with RAIT only (Wong et al. 2003).
While 90Y-cT84.66 was conjugated to isothiocyanatobenzyl DTPA (di-diethylenetriamine pentaacetic acid) during these first trials, data about a phase-I study in 90Y-DOTA-cT84.66 became first available in 2006 (Wong et al. 2006). In thirteen patients with advanced CEA-expressing tumors, dose-limiting haematopoetic toxicity of 90Y-DOTA-cT84.66 appeared to be comparable to previously published results for 90Y-DTPA-cT84.66 if Ca-DTPA infusions were given after RAIT in order to reduce circulating non–mAb-bound activity. Tumor dose estimates (4.4–569 cGy/mCi) and the HACA-incidence (8/13 patients) of 90Y-DOTA-cT84.66 were also comparable to those of 90Y-cT84.66.
4.1.2 Anti-CEA F(ab’)2-fragments in mCRC
The most challenging problem with RAIT is its inability to deliver a sufficient amount of radioactivity to destroy solid tumors (especially when >5 cm) at tolerable doses to other organs. Delivering radioactivity into the tumor has two major aspects: to concentrate enough radioactivity into the tumor and to distribute the radioactivity within the tumor homogeneously, so that all cancer cells receive a lethal dose of radiation. Therefore, anti-CEA F(ab’)2-fragments can be used with smaller molecular size compared to intact mAbs. The first clinical study in RAIT with anti-CEA F(ab’)2-fragments in CRC was published by Lane et al. (1994) testing the hypothesis that F(ab’)2 might achieve a more effective penetration and higher dosages into tumor deposits than intact antibodies. In this study, all patients (n = 19) had non-resectable, locally recurrent or metastatic malignancies (17 patients with CRC). The biodistribution of 131I-labeled F(ab’)2 was compared with its 131I-labeled parent antibody, the murine IgG1 anti-CEA mAb A5B7, using a MIRD and SPECT-based dosimetric approach. Although the uptake of the F(ab’)2-fragments in tumor deposits was higher at early imaging (4 h p.i.), late studies showed no significant difference in antibody uptake. More importantly, the cumulative dose per unit of administered activity to the tumor did not differ in both groups significantly. Two patients, one in the IgG group and one in the F(ab’)2 group, demonstrated partial clinical responses.
4.1.3 Anti-CEA mAbNP-4 (IMMU-4) in mCRC
The study investigated the pharmacokinetics, immunogenicity, and therapeutic effects of 131I-labeled F(ab’)2 fragments of the murine anti-CEA mAbNP-4 (IMMU-4, a class III CEA-specific mAb) in patients with small-volume (≤3 cm) CEA-expressing metastatic cancers. Within this study, eight patients with CRC were treated with repeated administrations of 131I-labeled NP-4. The activity of 131I was based on a prescribed radiation dose to the red marrow. Four CRC patients (50 %) showed stabilization of previously progressive disease for up to 7 months. Most patients (83 %) developed HAMAs after the second infusion.
Within another phase I/II-study (Mittal et al. 1996), the combination of 131I-labeled IMMU-4 (at 30 or 60 mCi/m2) with hyperthermia was also evaluated in six patients with CRC-LM. One patient experienced partial remission and two additional patients showed an improvement of their clinical symptoms. In five of the six patients, a decrease in serum CEA levels was observed.
Behr et al. investigated the anti-tumor efficacy of escalating dose-activity of 131I-labeled NP-4 in 57 patients with CEA-expressing malignancies (29 patients with CRC). The activity levels of radioiodine varied between 44 and 268 mCi. Minor anti-tumor effects were noted in 12 of 35 assessable patients (Behr et al. 1997).
4.1.4 Anti-CEA Murine MN-14 mAb in mCRC
The murine MN-14 mAb is a second-generation anti-CEA antibody with a ten-fold higher affinity (9 × 109 M−1) than the first-generation NP-4 mAb MN-14. This mAb has demonstrated superior tumor targeting in a human colon carcinoma xenograft model compared with the NP-4 antibody (Hansen et al. 1993). 188Re is an isotope with 17 h half-life decaying through beta emission, with a maximum energy and path length of electrons comparable to that of 90Y. A gamma emission at 155 keV makes the isotope suitable for imaging and dosimetry with gamma cameras equipped with a low-energy collimator. Juweid et al. (1998) evaluated the DLT and MTD and investigated on an outpatient basis the efficacy of 188Re MN-14 RAIT in 11 patients with widespread CEA-positive cancers (10 patients with CRC). Patients were treated with repeated administrations of 188Re-MN-14 at escalating dose levels, varying from 20 to 78 mCi. The MTD of fractioned doses of 188Re –labeled MN-14 was reached at 60 mCi/m2, with red marrow suppression being the only dose-limiting toxicity observed (Juweid et al. 1998). No therapeutic effect was observed in the patients. This observation may be related to the short physical half-life of 188Re (17 h). It can be assumed that in bulky and poorly vascularized metastases, a substantial proportion of the activity will have already decayed by the time the mAb has entered the tumor. A proper choice of a therapeutic nuclide that fits the kinetics of the antibody is therefore crucial.
4.1.5 Anti-CEA Humanized MN-14 mAb (hMN-14, Labetuzumab) in mCRC
Conventional RAIT using an intact IgG antibody subtype will be more successful in micrometastatic disease than in an advanced stage of cancer progression. Nevertheless, by using murine antibodies in RAIT, the development of HAMAs usually prevents repeated therapy and is therefore an important limitation of RAIT. One strategy to reduce the HAMA incidence after mAb-application is the development of humanized antibodies. Labetuzumab (hMN-14) was created by isolating only those regions from the MN-14 that define the antigen-binding site, i.e., the CDR and a minimal portion of the framework region that maintains the 3D structure of the CDR (Hansen et al. 1993; Sharkey et al. 1995). Thus, this humanized form of a subclass mAb retains only ~3–5 % of the original mouse residues, which is expected to make it less immunogenic than the murine mAb.
Radiolabeled Labetuzumab was demonstrated to target diverse tumors, including colorectal, pancreatic, lung, breast, and ovarian carcinomas and has similar biodistribution properties as the parent, murine anti-CEA antibody MN-14 (Sharkey et al. 1995).
To date, three reports have been published on RAIT using 131I-Labetuzumab in patients with mCRC. Behr et al. (1999a, b) and Hajjar et al. (2002) tested the toxicity and therapeutic efficacy of 131I-labeled humanized MN-14 in patients with mCRC. The former group focused on patients with small-volume CRC-LM (less than 2 cm), whereas Hajjar et al. (2002) included patients with gross metastatic disease of colorectal, pancreatic or gastric origin. The publication of Hajjar et al. is of special interest because all 21 patients received a diagnostic infusion of 131I-hMN-14 IgG (~8.0 mCi, 15 mg/m2) to study the pharmacokinetics, biodistribution, and dosimetry. One week later, 17/21 patients received a therapeutic infusion of escalating radioactive-doses of 131I-hMN-14 IgG. Blood pharmacokinetics and quantitative imaging were carried out again after the therapeutic dose. Radiation-absorbed doses to normal organs and tumors were determined by a MIRDOSE-3 algorithm.
Behr found the MTD of 131I-labeled hMN-14 to be 60 mCi/m2, with higher doses leading to Grade 4 hematologic toxicity. Hajjar et al. (2002) reported the MTD to be only 40 mCi/m2, which is in accordance to our own observations (Liersch et al. 2005, 2007) and the experience made with other second-generation anti-CEA mAbs (Chong et al. 2005). Hajjar et al. (2002) did not observe any tumor response, whereas Behr et al. reported three partial remissions and eight minor responses in a selected subgroup of 30 patients with metastatic disease.
In pseudoadjuvant intention, the Göttingen group was the first to report on the efficacy of RAIT used in nine patients with CRC-LM. Within 6 weeks, after R0 resection, Labetuzumab was administered at a single dose level of 60 mCi/m2. During a median follow-up of 27 months, only two patients had developed cancer recurrence (Behr et al. 2002). These preliminary but encouraging results were the basis for further phase I/II studies using 131I-labeled humanized MN-14 (Labetuzumab) in pseudoadjuvant intent in patient with CRC post-salvage resection of CRC-LM.
4.1.6 Pre-targeting Systems and Anti-CEA mAbs in mCRC
Pre-targeting systems are more promising than directly radiolabeled mAb as they are able to deliver a significantly higher total dose and an increased dose rate into the tumor with improved anti-cancer effects (Goldenberg et al. 2006; Sharkey and Goldenberg 2006). In general, the protocol for pretargeting was first described in 1989 as Affinity Enhancement System (AES). The basic idea behind the AES model was that divalent haptenes interacting with a bispecific mAb provide greater stability in the tumor than monovalent haptens. The primary model system assembled a Fab’ fragment from a murine anti-CEA mAb (F6) combined with a Fab’ fragment of an anti-DTPA antibody (mAb 734). The haptene used in early clinical studies was a di-DTPA peptide labeled with 111In for scintigraphy. Successful imaging procedures in CRC and medullary thyroid cancer using 1–10 mg of the bsmAb followed 2–8 days later with an 111In-labeled di-DTPA peptide were caried out. Scintigraphies showed low background activity, an excellent contrast and high resolution of the tumor sites (Le Doussal et al. 1993; Peltier et al. 1993; Vuillez et al. 1997). The group in Nantes reported data from a phase-I trial in 20 patients with CEA-expressing metastatic cancers (most of them with medullary thyroid cancers) treated with a pre-targeting system using a hMN-14 × m734 bispecific antibody (BsmAb) and 131I-di-diethylenetriamine pentaacetic acid (DTPA)-indium hapten (Kraeber-Bodéré et al. 2006). BsmAb dose of 40 mg/m2 and a 5-day interval appeared to be an optimal dose/schedule regimen with acceptable toxicity. Under these conditions, the maximal tolerated activity was 3 GBq of 131I-di-DTPA-indium in patients with medullary thyroid cancers. Beside hematotoxicity only mild hepatic toxicity (Grade 1 and 2) was noted. Nine cases of tumor stabilization up to 12 months were observed.
Anti-chelate antibodies are highly specific for the metal-loaded chelate, and therefore, this technology requires a new anti-hapten antibody in order to bind different radionuclides. To overcome these limitation, a novel pretargeting system that uses the compound histamine-succinyl-glycine (HSG-peptide) as the hapten and a bsmAb (TF2) based on the hMN-14 anti-CEA antibody and an anti-HSG antibody that has been recently developed (Rossi et al. 2006; McBride et al. 2006). TF2 is a 157 kD protein and binds divalently to CEA with the same avidity as the parental hMN-14 antibody and monovalently to the HSG-peptide. The HSG-peptide labeled with the positron emitter 68Ga for imaging (“Immuno-PET/CT”), and labeled with 131I for therapy is currently under investigation in phase-1 studies in patients with CEA-expressing tumors (Professor Oyen; Nijmwegen).
4.2 Colon-specific Antigen p and Chimeric B72.3 (Human IgG4) in mCRC
As previously described, several murine antibodies and antibody fragments directed against the CSA-p epitope have been produced in the early 1990s (Gold et al. 1990). In preclinical studies, one of these murine antibodies, the 131I-labeled Mu-9, showed advantages compared to 90Y- or 188Re-labeling and was subsequently used in human studies (Sharkey et al. 1997). Within an early phase-I trial, Sharkey et al. conducted a dose-escalation study in 25 patients with GI cancers (21 patients with CRC) to determine the maximum tolerated dose (MTD) for both the 131I-labeled IgG and F(ab)2 Mu-9. The dose absorbed by the normal organs (except the kidneys) was two- to threefold less for the F(ab)2 than for the whole IgG while the average tumor dose for 131I-Mu-9 IgG was two- to threefold higher than for the 131I-F(ab)2 fragment. The kidney dose was similar for both immunoconjungates. Most patients developed HAMAs, although levels in patients treated with 131I-F(ab)2 fragments were considerably lower compared with those in patients treated with the complete mAbs (Sharkey et al. 1994).
A number of 12 patients with CEA-expressing mCRC participated in a phase I trial using the 131I-labeled chimeric B72.3 (human IgG4). The dose-escalation levels were set at 18, 27, and 36 mCi/m2. Bone marrow suppression was the only significant side effect. The MTD was 36 mCi/m2 with all the six patients at this dose level having at least Grade 1 and two patients having Grades 3 and 4 hematologic toxicity (Meredith et al. 1992a).
In a second study, 12 patients with mCRC were treated at the same dose levels but the radiation activities were divided in two or three weekly fractions. Again after fractionated application of RAIT, the bone marrow suppression was the main side effect. Adjusted for the whole-body dosage, the degree of bone marrow depression was significantly (p = 0.04) lower than the identical dosages given as single infusions within the first phase I study. After fractionated RAIT, 9 of 12 patients developed an antibody response (HACAs), altering the antibody kinetics in subsequent courses of therapy and potentially decreased the tumor dose (Meredith et al. 1992b).
To overcome tumor heterogeneity and to allow delivery of high radiation dose, 131I-labeled COL-1 (anti-CEA mAb) and CC49 (anti-TAG-72 mAb) at a total dose of 75 mCi/m2 were concomitantly administered to 14 patients with mCRC cancer within a phase II study (Meredith et al. 1996). Additionally, interferon-alpha was given to increase CEA and TAG-72 antigen expression. Transient myelodepression and/or thrombocytopenia were the main side effects. In this study, no objective response was detectable (in four patients, stable disease; in ten patients, cancer progression) and the tumor dose estimates varied from 393 to 1327 cGy. The authors postulated that the amount of radiation delivered to tumor sites were still below that is required to induce tumor regression in patients with mCRC.
4.3 Anti-EpCAM 17-1A Antibody and Chimerics in mCRC
The anti-EpCAM antibody 17-1A is internalized in human colon cancer cells and can be used as a carrier of Auger electron emitters like 125I. In 1995, first results of a Pilot study in 28 patients with progressive mCRC treated with escalating doses of 125I-chimeric 17-1A have been reported. Although cumulative activities up to 250 mCi were used, no significant toxicity was reported (Meredith et al. 1995). No objective response was detected.
Renewed interest in EpCAM as a target in RAIT of CRC arose with the development of pretargeting technologies. Within a phase-II study, Knox et al. used a three-component pretargeted regimen (the streptavidin-conjugated murine anti-EpCAM, NRLU-10 mAb, followed by biotin-containing cleaning agents and 90Y -DOTA-biotin) to treat 25 patients with progressive mCRC. On day 1, all patients received NRLU-10 (Knox et al. 2000).
Approximately 48 h after NRLU-10 antibody application the clearing agent was administered. 24 h after application of the clearing agent, all patients received a single dose of 110 mCi/m2 of 90Y-DOTA-biotin. Grades 3 and 4 diarrhea was the most frequently non-hematologic toxicity and occurred in 32 % of the patients. The hematologic side effects resulted in Grade 4 neutropenia and thrombocytopenia in 8 and 16 % of patients, respectively. During follow-up, two patients demonstrated detoriation of renal function 7 and 8 months after treatment. The overall response rate to RAIT in these 25 patients with advanced mCRC was low (8 %). However, in this study, the most convincing explanation for the described toxicity resulted in binding of NRLU-10 on normal GI epithelium and in renal collecting tubules combined with renal retention of the radiolabeled haptenes (Knox et al. 2000).
4.4 Anti-TAG-72 Antibodies in mCRC
The murine mAb CC-49 is characterized by a six-fold higher affinity (1.62 × 1010 l/mol) for the TAG-72 antigen compared with the chimeric B72.3 (human IgG4). Murray et al. treated 15 patients with chemotherapy-refractory metastatic colon cancers by using 131I-CC49 to determine the toxicity and clinical efficacy of this RAIT. All patients received 75 mCi/m2 131I-CC49 intravenously. At 4–5 weeks after treatment, reversible hematologic side effects like Grades 3–4 thrombocytopenia and Grades 3–4 granulocytopenia were observed in 46 % and 40 % of all patients, respectively. During short-time follow-up (5 weeks), 92 % of the patients developed HAMAs. In two of three patients, treated a second time, HAMA-induction led to an increased whole body clearance rate and reduced uptake in the tumor. No clinical cancer-specific response was observed in this trial with mumAb CC-49 (Murray et al. 1994). In another study, performed by Divgi et al. (1995), 24 patients with TAG-72-expressing CRC were treated with escalating dosages of 131I-CC49 starting at 15 mCi/m2 up to 90 mCi/m2 131I-CC49. After completion of therapy, four weeks later, there was a stabilization of disease detectable in six patients. Additionally, a minor response occurred in one patient receiving a second application of 131I-CC49. Within a pilot study on TAG-72 positive cancers, Mulligan et al. treated three patients with mCRC using the 177Lutetium labeled (DOTA)-conjugated CC-49 mAb. The rationale for the use of 177Lu was that this lanthanide with a physical half-life of 6.7 days offers both an intermediate energy emission and range β emission (average energy of 133 keV, maximum energy of 497 keV) penetrating 0.2–0.3 mm into soft tissue. 177Lu also emits two relatively low-abundance, low-energy γ rays (113 and 208 keV) that allow imaging with a gamma camera. In the mentioned Pilot study, an unexpected uptake of the radiopharmacon into the bone marrow resulted in Grade 4 hematologic toxicity. The MTD was limited to only 15 mCi/m2 (Mulligan et al. 1995).
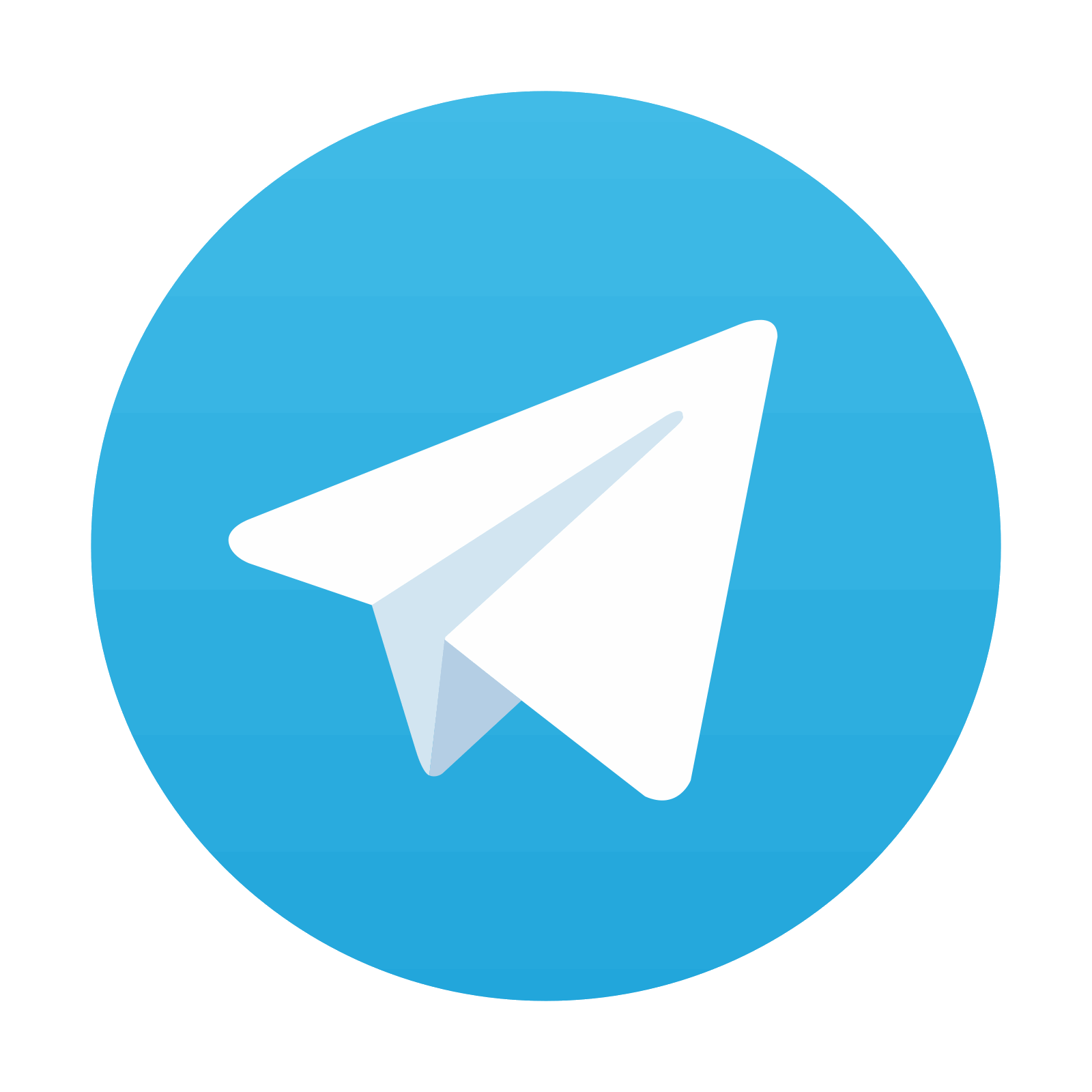
Stay updated, free articles. Join our Telegram channel
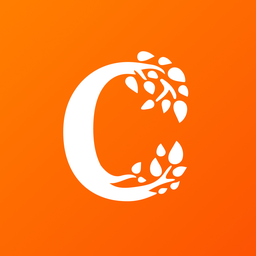
Full access? Get Clinical Tree
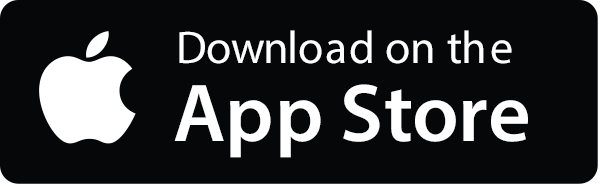
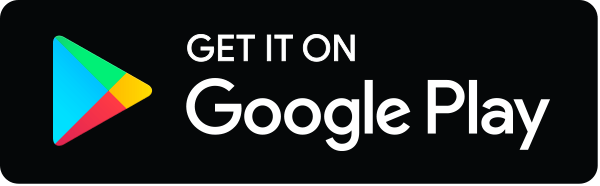