Fig. 16.1
Historical overview of development of optical imaging into the clinic. The first contrast agents were used by Moore et al. between 1940 and 1950. Other milestones of optical imaging in surgery were the introduction of a blue dye in localizing the sentinel lymph node in breast cancer treatment (1994). The introduction of 5-ALA in brain surgery (1998). The more recently, the use of folate receptor-alpha-FITC in human ovarian studies (2011)
The need to advance the imaging technology employed in operating rooms stems from the limitations of human vision and touch. The human eye cannot visualize beyond the tissue surface, and moreover it lacks sensitivity to molecular-based features. Separating tumor tissue from surrounding healthy tissue is a challenging task which cannot be optimally carried out by human vision or palpation; the latter also employed as means to identify tumor tissue based on different elasticity characteristics that may be present between healthy and malignant cell formations.
Although tactile information is considered by many surgeons as an important feature in staging, the method is not ideal for detecting small volumes of disease and clusters of few cells; the sensitivity, specificity, and diagnostic accuracy of palpation in cancer surgery lack currently thorough evaluation and corresponding literature. As a result the most accurate method of evaluating the success of, for example, a surgical oncology procedure is pathology, which although serving as the gold standard is typically applied postoperatively in a delayed fashion. Due to technical limitations good and complete pathological examinations may take up to 4–7 days. In this case, confirmation on the surgical outcome and importantly a prognosis, results come while the patient is in recovery, which limits opportunities for further intervention compared to achieving such diagnostic capacity immediately during surgery.
In the ideal situation, an imaging modality can assist a surgeon by giving direct real-time information and feedback on otherwise invisible disease biomarkers, while it enhances the detection sensitivity and specificity over palpation of visual inspection. This information should be available in the operating room, without requiring post-processing or elaborate data analysis, so that it can directly impact decision making during intervention. These approaches may improve surgical outcome, decrease operation time and importantly might improve the prognosis of the patients.
The emergence of fluorescence-based video-rate methods that can enhance disease detection in macroscopic inspection of the field of view available to the surgeon or endoscope employed promises to revolutionize surgical procedures. The use of nonspecific fluorescence dyes, such as fluorescein, was considered for this purpose more than 60 years ago [1]. Since then different nonspecific dyes but also progress with targeted agents that reveal molecular biomarkers of cancer cells promise to improve the theranostic ability during surgery. Porphyrins [2, 3], indocyanine green (ICG) [3], fluorescein-albumin [4], nontargeted fluorescent nanoparticles [5], and viral replication [6] strategies have been considered for tumor margin delineation [2, 5]. In addition lymph node identification/staging [7, 8], neuronal activity monitoring [9, 10], nerve imaging [11], bile duct imaging [12], and vascular mapping [13] have also been considered. Overall, fluorescence imaging comes with significant advantages over human vision and possibly over other optical imaging techniques.
The use of fluorescence imaging should be considered together with accurate imaging methods to ensure quantitative fluorescence inspection, in particular methods that correct for the effect of optical properties on the fluorescence signals. In the following chapter, the basic principles of optical imaging are illuminated, focusing on how optical imaging relates and fits to the operating room. Promising directions and applications are also discussed.
Surgical Optical Imaging
Currently, an array of imaging modalities is preoperatively applied to gain insights on disease staging and to recommend a combination of chemotherapy, radiotherapy, and surgery [14–22]. With the advancement of noninvasive radiological modalities for preoperative tumor staging, such as magnetic resonance imaging (MRI) [15, 16, 20], computed tomography (CT) [23], and positron-emission tomography (PET) [19, 22], the prospects for preoperative staging and therapeutic decision making have markedly improved in the last decades and can be used to better define selected patient groups.
Despite their preoperative benefits, established radiological modalities are not ideally suited for applications in the operating room due to the following reasons:
1.
Most radiological modalities are of size and weight that make them impractical for use in confined spaces such as the area around the operation table.
2.
Most radiological modalities do not allow easy access to the patient since they require that the patient resides inside the scanners bore for imaging purposes.
3.
High resolutions achieved by common radiology modalities (e.g., 1 mm when using a CT scan) may be considered as stellar for whole-body imaging, but they are very low compared to the human vision (50 μm) and the ability of optical methods (<1 μm) when considering surgical fields of view.
4.
During surgery real-time feedback is needed, and the field of view should relate to the surgeons vision, none of the abovementioned modalities are able to give these essential properties during a surgical procedure.
Despite the engineering of open-bore systems and scanners of smaller dimensions, the above limitations remain for most radiological systems. In contrast, portable or handheld systems are more appropriate for imaging applications. In this area ultrasound, portable high-energy (ionizing) detectors, and optical methods qualify as more practical alternatives for intraoperative imaging. Each of the methods comes with its own advantages and limitations. Ultrasound can reach relatively high resolution when considered in specialized implementations (up to 30 μm or even better) and can see depths of several millimeters to centimeters depending on the resolution (frequency) achieved. Conversely, its field of view is limited, and it further requires contact with tissue, which is impractical in many surgical applications. Gamma detectors can be used to detect deep-seated disease and can be very useful to image guidance; however, continuous use of radioisotopes may increase the occupational risk for surgeons and surgical personnel, and the resolution achieved with these methods does not compare with that of human vision or optical methods. Optical imaging therefore presents interesting features, such as the use of nonionizing radiation, high resolution, portability, and cost efficiency, and an excellent relation to the human vision. Although depth penetration cannot practically reach beyond 1–2 cm due to absorption and scattering of photons through tissue [24], superficial activity can be detected with high sensitivity. Therefore its use in surgical environments has merit, and certain of its features are further elaborated in the following.
Basic Principles of Intraoperative Fluorescence Imaging
The human eye can detect information in the visual spectrum ±400–750 nm (Fig. 16.2) with a resolution of approximately 50 μm. While human vision is unparalleled in detecting and understanding shapes and architectural features, the human eye is not a great spectral detector. For example, it is difficult for the human eye to differentiate objects with similar colors. For this reason, the army commonly use camouflage closure to conceal soldiers and structures, while camouflage does not have the same chemical composition and the identical color as the surrounding environment it nevertheless results in misleading the enemy’s eye. It is similarly difficult for the surgeon to detect discolorations, and it is virtually impossible to sense biochemical changes between healthy and malignant tissue with human vision. Molecular changes are generally invisible to the naked eye. For this reason, benign scar tissue or tumor margins and locoregional metastasis cannot be easily visualized during surgery.
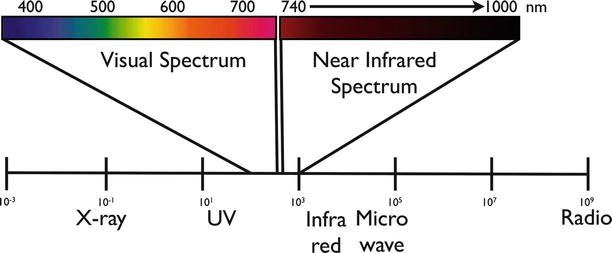
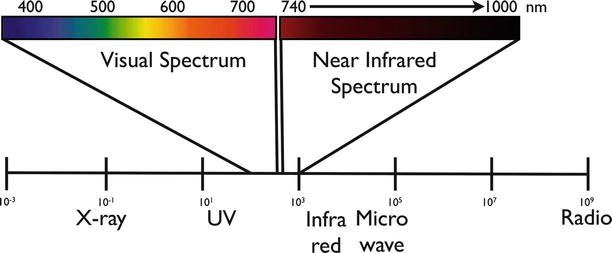
Fig. 16.2
The visual spectrum (400–750 nm) and the near-infrared spectrum (750–1,000 nm) highlighted in the light spectrum. NIR is not visible by visual observation and can only be detected by sensitive camera systems
Moreover, light in the visible wavelength hardly penetrates into tissue. Near-infrared (NIR) light around 750–1,000 nm penetrates deeper (Fig. 16.3) because hemoglobin, the principle absorber of visible light in the visible, water, and lipids offer lower absorption of light in this spectral region. Another advantage of NIR fluorescence imaging is that the autofluorescence of the tissue is low, which can improve substantially the target to background ratio over imaging in the visible [25]. A target to background ratio (TBR), often calculated as the ratio of signal coming from the malignant lesion or the lesion of interest over signal from determined locations considered as background tissue (such as muscle or skin), is a common metric for calculating the contrast of an imaging modality. Obviously a diagnostic or theranostic modality performs better when clear contrast (high TBR) exists between the target (i.e., tumor, lymph node, nerve, vessel) and background tissue.
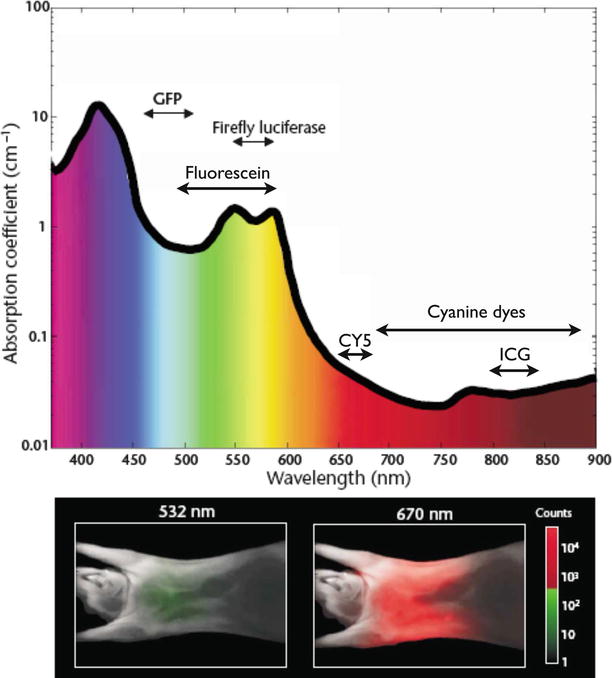
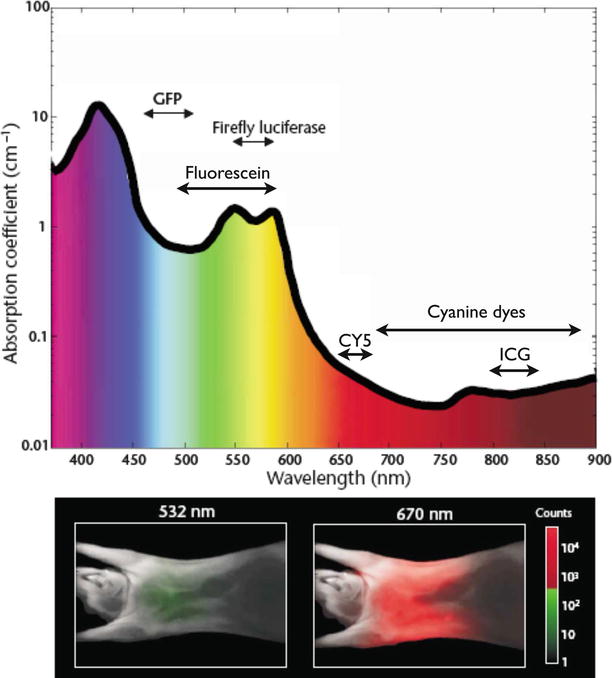
Fig. 16.3
Interaction of light with tissue. The absorption coefficient of light in tissue is dependent on wavelength and results from absorbers such as hemoglobin, lipids, and water. The graph is calculated assuming normally oxygenated tissue (saturation of 70 %), a hemoglobin concentration of 50 mM, and a composition of 50 % water and 15 % lipids. The graph also lists the emission range of several common fluorochromes and luciferases used for imaging. The mouse images at the bottom show experimentally measured photon counts through the body of a nude mouse at 532 nm (left) and 670 nm (right). The excitation source was a point illumination placed on the posterior chest wall. Signal in the NIR range is ∼4 orders of magnitude stronger for illumination in the NIR compared with illumination with green light under otherwise identical conditions, illustrating the advantages for imaging with NIR photons (Adapted with permission from Macmillan Publishers Ltd: Weissleder and Ntziachristos [25], copyright (2003))
While several optical methods could be considered for intraoperative imaging, such as confocal microscopy, two-photon microscopy, or optical coherence tomography (OCT), fluorescence imaging has important features to be considered first. The most important is that a sensitive video camera can inspect the entire surgical field and obtain a complete picture of the tissue and foci of possible malignancy. Fluorescence is an optical phenomenon in which the molecular absorption of photons (denominated as excitation) at a certain wavelength or wavelength range triggers the release of other photons in a different (longer) wavelength range (denominated as emission).
The source often used for excitation is a light beam, for example, a filtered white light beam or a laser beam, illuminating the field of view. The light is absorbed by the fluorescent probe which is then “activated” to release photons in a different wavelength that will occur. Fluorescence in this case is emitted from within the tissue that contains such fluorescent dyes and can be detected by a special camera system.
Camera systems used for intraoperative optical imaging consist of various components; an example is given in Fig. 16.4. A system should contain one or two light sources for excitation of the field of view (color and fluorescence or only fluorescence). The emitted light from the field of view is then guided through optics, and it is generally spectrally separated to color image, a fluorescent image, and possibly other images of different spectral content. Wavelengths of interest are separated by dichroic mirrors or filters and guided to the different cameras. Typically such system will use different types of cameras. For example, a highly sensitive charged-coupled device (CCD) camera(s) can be used for fluorescence detection, whereas color cameras are used for providing the color images. Computer software can then collect and process this data, potentially correct it for the effect of optical properties and autofluorescence, and render it alone or as hybrid images, for example, fluorescence images/video merged with the color images/video.
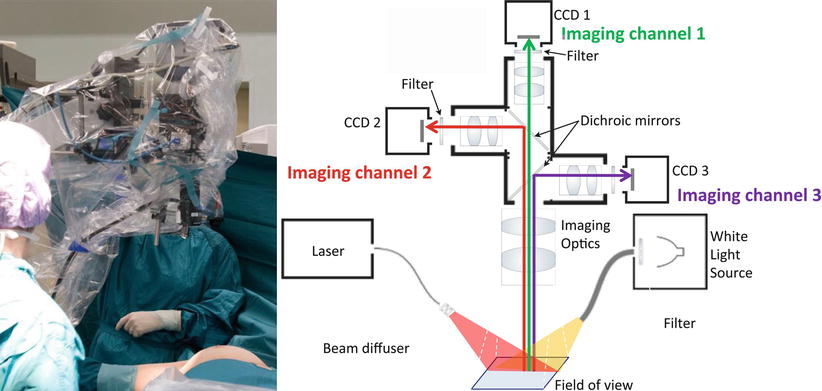
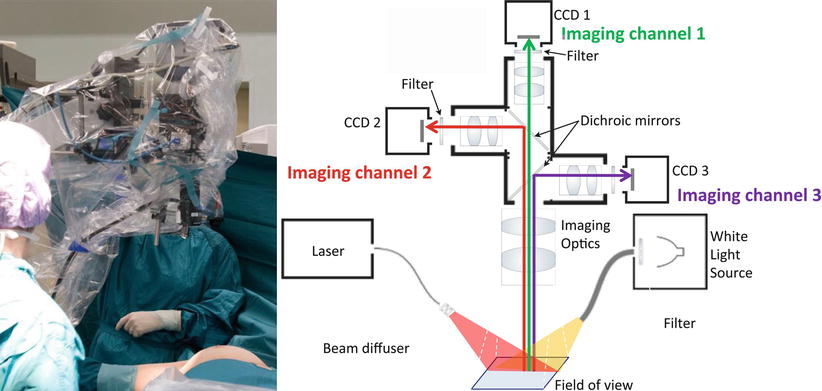
Fig. 16.4
Left: multispectral fluorescence camera system in the operating theater. Right: schematic of a multispectral fluorescence camera system, capable to capture simultaneously, in real-time three imaging channels: color reflectance, fluorescence, and intrinsic (excitation). A halogen light source is used for white light illumination and a diode laser for fluorescence excitation (From van Dam et al. [29])
An example of a camera system used in clinical studies [26–29] is shown on Fig. 16.4. The system consists of three CCD cameras for three different signals, i.e., NIR absorption (attenuation), NIR emission (fluorescence), and color imaging, collected simultaneously. The use of the absorption image is a particular feature of this system as it captures spatial variations of excitation light and can be used to correct the fluorescence image for heterogenous illumination artifacts [30, 31]. This is needed since single planar fluorescent imaging can give images of false negative or false positive if not corrected for optical attenuation [32].
This is a practical feature for OR or endoscopy systems that dynamically interact with three-dimensional tissue structures, often distorting the illumination homogeneity and differential light absorption by tissue due to varying illumination angles, modification of the intensity delivered, and the spatial heterogeneity of tissue absorption and scattering. The prototype camera systems are relatively bulky, but the technique offers to be build in handheld, very compact systems like laparoscopic and endoscopic instruments.
NIRF Probes (Fig. 16.5)
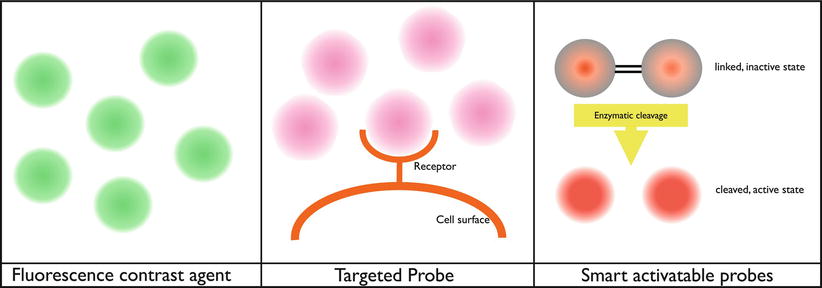
Fig. 16.5
Schematic working mechanism of fluorescent probes. Left: contrast agent, the probe is fluorescent by itself (after illumination); depending on the place of injection, it will give a contrast to the structure. For example, when administered to the circulation, it will give fluorescent contrast in areas with vascularization. Middle: targeted probe, the probe is fluorescent by itself (after illumination) and will attach to receptors on the cell surface of interest. For example, folate receptor-alpha in combination with FITC. Right: smart activatable probe, the probe is nonfluorescent by itself but will be fluorescent after enzymatic cleavage (after illumination). These kinds of probes need to be injected intravenously. When the probe passes the region of interest, it will be cleaved by enzymes, and the probe becomes fluorescent. For example, the commercially available probe MMPSense (PerkinElmer, Massachusetts, USA) that is activated by matrix metalloproteinases (MMPs)
Nonspecific Fluorescent Probes/Contrast Agents
In radiology, contrast agents are employed to enhance different tissue structures. Iodine-based contrast agents for X-ray or gadolinium-based agents for MRI operated by enhancing tissues based on physiological parameters, such as perfusion and permeability differences between different organs. The equivalent of such agents for optical imaging includes methylene blue, fluorescein, and indocyanine green (ICG) employed to visualize structural or functional characteristics of tissue, such as retinal angiography, hepatic clearance, and outline lymph nodes, and investigate blood perfusion in various organs. By operating in the near infrared (805 nm ex.; 835 nm em), ICG is also suitable for deeper tissue applications [25, 33], compared to fluorescein which has maximum absorption at 494 nm and maximum emission at 591 nm. This is because, as already explained, near-infrared wavelengths are attenuated significantly less by tissue compared to visual wavelengths. Several centimeters of tissue penetration have been achieved in transillumination mode, for example, more than 10 cm in breast tissue [33, 34]. However in epi-illumination applications, as appropriate for interventional imaging, 2–3 cm is a more realistic depth assuming higher absorbing tissues compared to breast tissue. ICG and FITC are currently approved for clinical use by the Food and Drug Administration (FDA) and the European Medicines Agency (EMEA).
Targeted Probes
Fluorescently labeled antibody, antibody fragments, peptides, and minibody-based probes have a high potential to improve intraoperative and endoscopic imaging by increasing the sensitivity and specificity over the detection capacity of human vision. Probes with targeting capacity of disease biomarkers can enable therefore imaging of otherwise invisible molecular processes associated with disease formation and growth. Several new fluorescent probes have been introduced over the past decade, enabling the capacity to target major biomarkers in cancer, inflammation [35], neurodegenerative diseases [36], cardiovascular diseases [37, 38], and so on.
Generally the introduction of a new fluorescent tumor-specific probe for clinical use is challenging and has to overcome important regulatory and safety concerns. For this reason clinical translation of such agents, while a potent strategy, has been slow. However, the clinical translation of a folate-targeting agent has been achieved, demonstrating significant potential for the use of fluorescence molecular imaging in interventional procedures [29] (see also examples in the following). Overall, theranostic agents can be based on many different chemistries, including labeling of existing drugs; in analogy to nuclear-imaging studies labeled bevacizumab, for example, an antitumor drug targeting VEGF in several types of cancer has been conjugated to a PET radionuclide and is used for preoperative visualization of tumors [39]. Similarly, the conjugation of IRDye® 800CW (LI-COR® Biosciences) [40] a fluorescent dye was achieved and demonstrated the ability to visualize tumors in mouse xenografts with high sensitivity and specificity (Fig. 16.6). This paradigm can be extended to many possible therapeutic molecules considered so far.
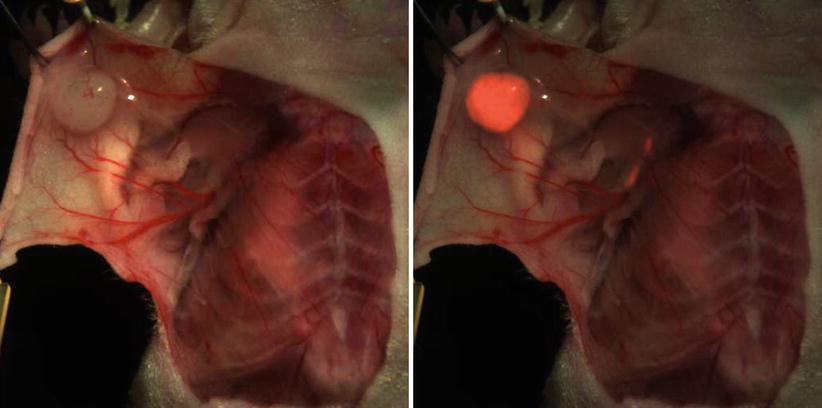
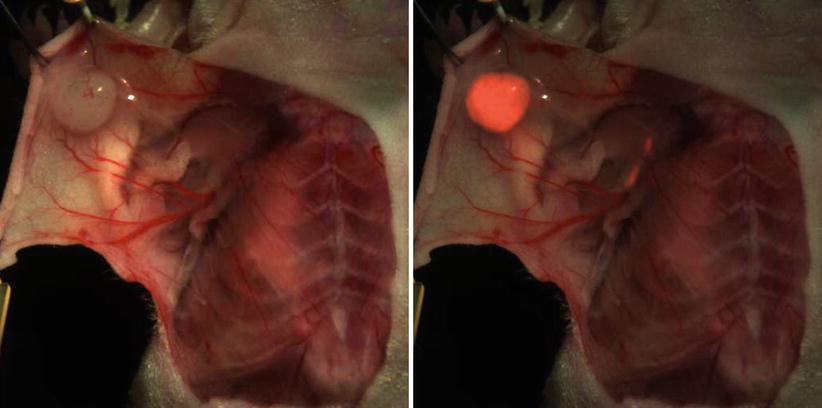
Fig. 16.6
Example of a murine breast cancer model. Color imaging and fluorescent overlay 24 h after injection of 800CW-bevacizumab
Overall, a new fluorescent probe needs to achieve favorable biodistribution and clearance, no measurable toxicity, efficient disease targeting, and high target to background ratio. Utilizing fluorochromes in the near infrared comes with the added advantage of improved detection sensitivity. Other optimal characteristics include high quantum efficiency and sensitivity to physiological parameters, most notably pH.
Activatable Probes
Another class of fluorescent probes with high molecular specificity is the so-called smart probe concept. The underlying mechanism is explained in Fig 16.5. Typically, a macromolecule is loaded with multiple fluorochromes, attached with linkers that are enzymatic substrates to specific enzymes. The fluorochromes in this case self-quench due to proximity [28]. However, after enzymatic cleavage (e.g., cathepsins, matrix metalloproteinases (MMPs), elastases, and proteinases) the probes are detached from the macromolecule and are free to fluoresce. Therefore interaction with specific enzymes leads to “probe activation” which has the potential to suppress background “nonactivated” probe distribution. Conversely, free fluorochromes may redistribute however and reduce TBR.
This example design has many alternatives and can include small molecules containing a fluorochrome and a quencher or enzymatic digestion of the macromolecule and not the linker (enzymatic substrate) between the macromolecule and the fluorochrome.
Intraoperative Optical Imaging, from Bench to Bedside
The translation of targeted and activatable probes in clinical settings may significantly enhance surgical vision. Translation is an elaborate process that contains significant risk regulatory aspects, administrative procedures with complicated filing procedures, and is a slow process. Despite the barriers to clinical entry, many studies are geared towards demonstrating the interventional use of such agents, which may be used as the stepping stone for providing evidence on the efficacy and safety of a fluorescent agent. Examples are included in the following that outline the potential of intraoperative fluorescence molecular imaging.
Detection of Positive Resection Margins in Breast-Conserving Therapy
In order to avoid the mutilating procedure of a complete mastectomy in breast cancer, modern breast-conserving surgery (BCS) is commonly applied and consists of two steps. An initial lumpectomy is followed by irradiation of the breast. To achieve the best balance between optimal cosmetic results and preservation of negative resection margins, accurate intraoperative localization of the tumor is essential for adequate surgical removal in a one-step procedure. In BCS, positive resection margins are reported in 20–40 % of all patients [41], ranking up to 65 % [42].
This may lead to additional surgery and even increased mastectomy procedures at a later stage. Additional surgery comes with increased risk, psychological distress for the patient, and a decreased cosmetic result. Several new imaging techniques have been introduced to reduce the number of positive resection margins. Pleijhuis et al. [43] describe the advantages and disadvantages of the following imaging techniques in order to obtain a negative resection margin: MRI for improved staging preoperatively, (wire) guided localization for small tumors, ultrasound-guided resection, and a direct postoperative specimen radiography. All these methods assist in localizing of the tumor but do not give intraoperative real-time feedback on the resection margin. Frozen section analysis on the excised specimen delivers this feedback on resection margins but is not real time and time consuming, and its reliability on negative margins is questionable due to a high variance in diagnostic sensitivity [44].
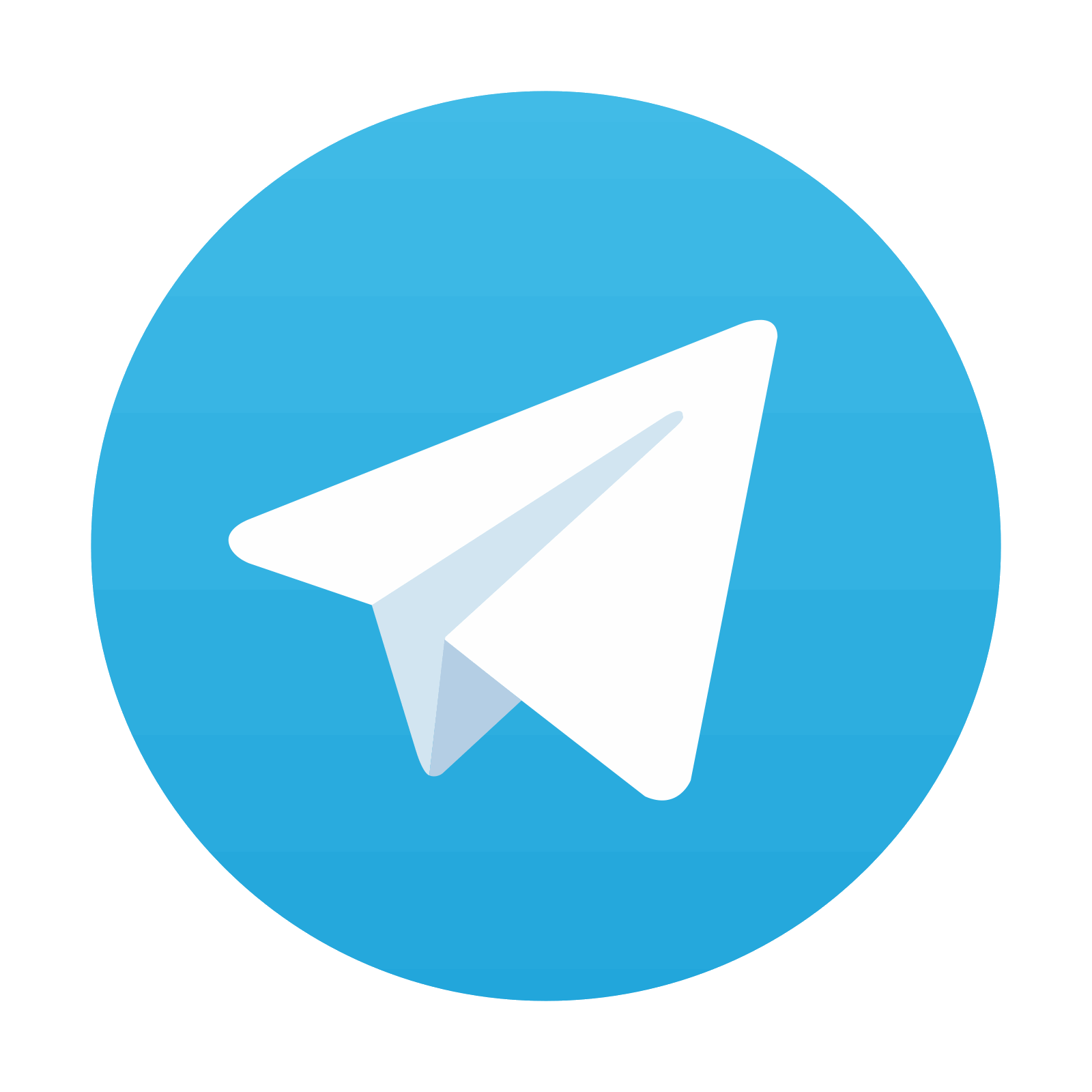
Stay updated, free articles. Join our Telegram channel
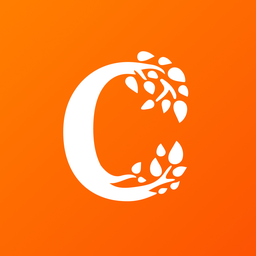
Full access? Get Clinical Tree
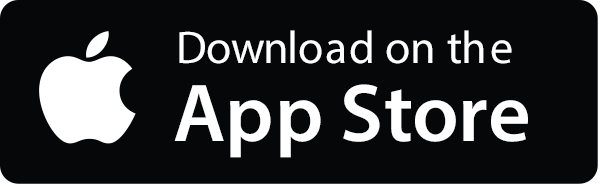
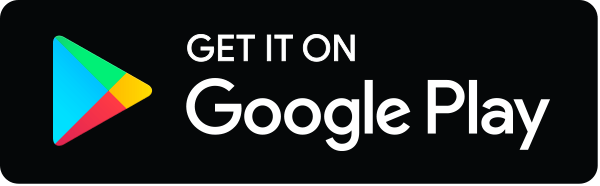