(14.1)
Absorbed dose (D) is the amount of energy (J) that is deposited in a unit mass (kg), and is represented by the SI unit Gray (Gy). Although the unit is the same as KERMA (the gray: Gy), D is the energy deposited in a volume; while K is the energy transferred to charged particles (i.e. electrons) in that volume. In the diagnostic energy range D≈K, however as photon energy increases, K will grow substantially larger than D, thus this approximate relationship is only valid at low photon energies, such as those used in diagnostic imaging (<150 keV).
Neither of the two previous units account for the biologic effect of ionizing radiation. Equivalent Dose (H) is the first of two units that account for the relative biological effect (RBE) of the same energy deposition in tissue/cells due to the type of radiation administered (e.g. photons (x-ray, gamma ray), electrons, protons, etc.). To calculate H, D is scaled by a radiation weighting factor dependent on the radiation type. In diagnostic imaging H=D≈K since the RBE weighting value for photons is one. H is measured in SI units by the Sievert (Sv).
Effective Dose (E) accounts for the differing radiation sensitivities of organs within the body. It was previously referred to as “effective dose equivalent” and is defined as a weighted sum of the equivalent dose (H) to all exposed organs. The organ specific weighted sum coefficient incorporates the radiosensitivity of the organ, while equivalent dose (H) incorporates the RBE for the radiation type. E is defined mathematically in Eq. 14.2 and is measured in SI units by the Sievert (Sv).

Of the metrics described, E is the only metric that is related to the stochastic risk to the population and allows for risk comparisons between different sources of patient radiation exposure (e.g. different x-ray imaging examinations, nuclear medicine, natural background, air travel, etc…). In practice the E calculation is complex, and is neither practical nor designed for an individual patient risk assessment [5]. The calculation and risk associated with E is based on an exposure to a 30 year old, 70 kg “Reference Person.” Risk is estimated by linear extrapolation of higher dose data at an increased cancer fatality rate of 5.5 persons per 100,000 people per mSv exposure.

(14.2)
Given the wide application and specific geometry of CT scanners, three CT specific dose metrics have been developed. These include the CT Dose Index (CTDIw/vol), Dose-Length Product (DLP) and Size Specific Dose Estimate (SSDE).
CTDIw/vol represents the x-ray energy absorbed in a Polymethyl Methacrylate (PMMA) phantom with a standard diameter (Body: 32 cm, Head 16 cm) and is measured using the milli-gray (mGy). The subscript “w” denotes a weighted average of the dose at the phantom centre and periphery over 1 gantry rotation measured using a 100 mm pencil chamber in an trans axial acquisition. The “vol” subscript denotes the x-ray energy absorbed in a volume of PMMA from a helical acquisition, and is simply the CTDIw divided by acquisition pitch (defined below) [6, 7].
CTDIw/vol has been designed to be easily and reproducibly measured by Medical Physicists in routine CT quality assurance programs. In North America this measurement is A required radiation dose metric in quality control [8–10]. In practice it ensures individual scanner radiation output is within specification. This metric can also be used to compare radiation output between scanners and provide guidance for the exposure effect of CT acquisition parameter adjustments.
DLP is the product of CTDIw/vol and scan length (cm), with unit’s mGy-cm and represents the dose delivered to a scanned volume. It is very important to note that DLP does not represent the dose to the patient – it only represents the dose delivered by the CT scanner to a standard PMMA phantom of a standard diameter with the scanner parameters employed [11]. However, E can be roughly estimated by multiplying the DLP by a conversion factor for a standard reference patient. It is generally accepted that the E calculation using this method can be off by a factor of 3 or more, and is therefore unsuitable to be used as a patient specific dose. DLP in concert with CTDIw/vol are useful metrics in developing Diagnostic Reference Levels (DRL’s), which can be used to compare radiation usage for different protocols on different scanners or hospitals, as well as estimate the radiation received to the population from CT.
Size Specific Dose Estimates (SSDE) is a dose indicator established by the American Association of Physicists in Medicine (AAPM) Task Group 204 in 2011 [12]. As previously described, the CTDIw/vol represents the dose output of the scanner for a standard 32 cm or 16 cm phantom. It does not incorporate patient specific body habitus variations in patient size. SSDE incorporates the patient’s water equivalent diameter and scanner pre-hardening filter to improve the estimate of dose absorbed by the patient beyond CTDIw/vol. As stated by the AAPM for pediatric patients, interpreting the displayed CTDIvol (or DLP) as patient dose – without recognizing the distinction between the two – could lead to underestimating patient dose levels by a factor of 2–3 if the 32 cm PMMA phantom is used as reference (ref AAPM 204). SSDE is calculated using the patient’s dimensions (AP, LAT, AP+LAT or effective diameter) and a conversion factor based on patient size and the pre-hardening filter used by the system during image acquisition (32 cm or 16 cm). SSDE improves patient specific dose estimation from a factor of 2–3 (using CTDIw/vol) to within 20 %; however at current writing there is no established method to convert SSDE to neither a DLP-equivalent metric nor E. This work is currently under investigation by the AAPM.
Radiation Risk
The Linear No-Threshold Model (LNT) is the accepted risk model for the induction of cancer from low levels of ionizing radiation. This model states that the stochastic risk of a fatal (solid) cancer occurring in the population increases linearly with effective dose. As such the statement “at no dose is there no risk” is used to describe the risk associated with low levels of ionizing radiation.
Despite over 100 years of research, the biological effects at low levels of radiation remain a controversial topic. The definition of what threshold constitutes “low levels of radiation” is not universally accepted by national and international bodies. For example, the Biological Effects of Ionizing Radiation (BEIR) set 100 mSv as the limit for low level radiation effects [3], while the United Nations Scientific Committee on the Effects of Atomic Radiation (UNSCEAR) set this limit at 200 mSv [13]. Some researchers believe in “Radiation Hormesis,” a hypothesis which stipulates that exposure to low levels of radiation may lead to improved health and reduced cancer risk via up-regulation of cellular repair mechanisms that are initiated by exposure. This phenomenon has been demonstrated in a laboratory setting; however no scientific proof of this phenomenon has been shown in vivo. At the opposite spectrum of Radiation Hormesis is the “Radiation Induced Bystander Effect,” which stipulates that non-irradiated cells can exhibit characteristics of an irradiated cell, if they come into contact (i.e. the same medium) as cells irradiated during exposure or come into contact with damaged cells after exposure. The bystander effect proposes that low levels of radiation maybe more damaging then estimated with the linear no threshold model.
The biological risk for solid cancers induction by low level ionizing radiation exposure is described by the linear no-threshold model (LNT). As noted previously, the threshold for low level exposure varies between documents, being 100 mSv for Biological Effects of Ionizing Radiation (BEIR) and 200 mSv for the United Nations Scientific Committee on the Effects of Atomic Radiation (UNSCEAR). The LNT model was primarily developed using data from the Life Span Study (LSS), which followed the atomic bomb survivors of Hiroshima and Nagasaki. Estimates for the LNT model coefficients have been driven by radiation exposure >500 mSv, although the median exposure was 100 mSv. Multiple epidemiological studies of cancer patients analyzed by national and international bodies support the LNT model for risk estimation of low levels of radiation. Revised epidemiological data has shown elevated risk to persons irradiated >100 mSv [13]. Below 100 mSv the statistical power of a study is greatly affected by sample size and the relative risk for a statistically significant study is likely to be an overestimate of “true” risk [14].
Although the LNT model is currently supported by various national and international bodies the mechanistic effect of DNA double strand breaks and the resulting cancers have raised questions regarding the validity of the LNT model [15]. Without digressing into the intricate details of radiobiology, which is beyond the scope of this chapter, the LNT model appears to be the best risk estimate we can produce based on the data available [16].
Since radiation is classified as a weak carcinogen, the radiation safety community has established the ALARA principle, that is – As Low As Reasonably Achievable. Using the lowest radiation dose possible to achieve diagnostic quality images ensures we mitigate the currently unknown risk of radiation at low radiation levels, while enabling clinicians to obtain the benefit of adequate diagnostic quality images to guide clinical management. Using the LNT model with age and sex adjustments and epidemiologic data on mortality risk from suspected clinical conditions, a simple ratio of benefit of avoiding mortality from pulmonary embolism to the Lifetime Attributable Risk (LAR) of fatal radiation induced cancer from the radiation administered in a pulmonary embolism protocol CT scan can be calculated [17]. Woo et al. showed the benefit-risk ratio is strongly in favor of clinically indicated CTPA examinations for both men and women from ages 15 to greater than 80 [17]. However, since radiation risk is linear with radiation dose, dose reduction with preservation of diagnostic image quality is important for all CT exams.
Current radiation risk models have identified age and gender of the patient at exposure as important influences of developing radiation related effects [3]. For uniform whole body exposures younger patients are more susceptible to radiation related effects, as are females compared to males at the same age [18]. Risk decreases with increasing age, being very low above the age of 80. Up to the age of 60, females are more sensitive to radiation than males of the same age. Although this effect is partially modulated by breast tissue in females up to the age of 50, above this age breast tissue is relatively radiation insensitive and the chest organs at most risk in both males and females are bone marrow and lung [1]. Current models indicate that the onset of radiation induced solid cancers maybe 20–30 years after exposure [19]. Given the latency of radiation induced solid cancers, older patients may die from other competing disease processes (i.e. smoking, diet, obesity, diabetes, etc…) prior to expressing a potential radiation related effect.
In medical imaging radiation exposure is localized to the anatomically region of interest and is therefore not uniform. The risk estimate from CT is calculated by converting the Dose Length Product (DLP) to effective dose (E) using a conversion factor specific to the anatomical region examined (i.e. head, head & neck, trunk, etc…) [20]. This approach only partially accounts for organ-specific radiosensitivity via ICRP 103 weighting factors [5]. As demonstrated by Ivanov et al. calculating Lifetime Attributed Risk (LAR) using E instead of organ specific models from the ICRP 103 can lead to an underestimation of risk (in their example use of E lead to a 1.73-fold reduction in LAR) [21]. To improve LAR estimates it is advisable for authors to utilize the latest ICRP or BEIR organ-specific models, explicitly stating which model was used.
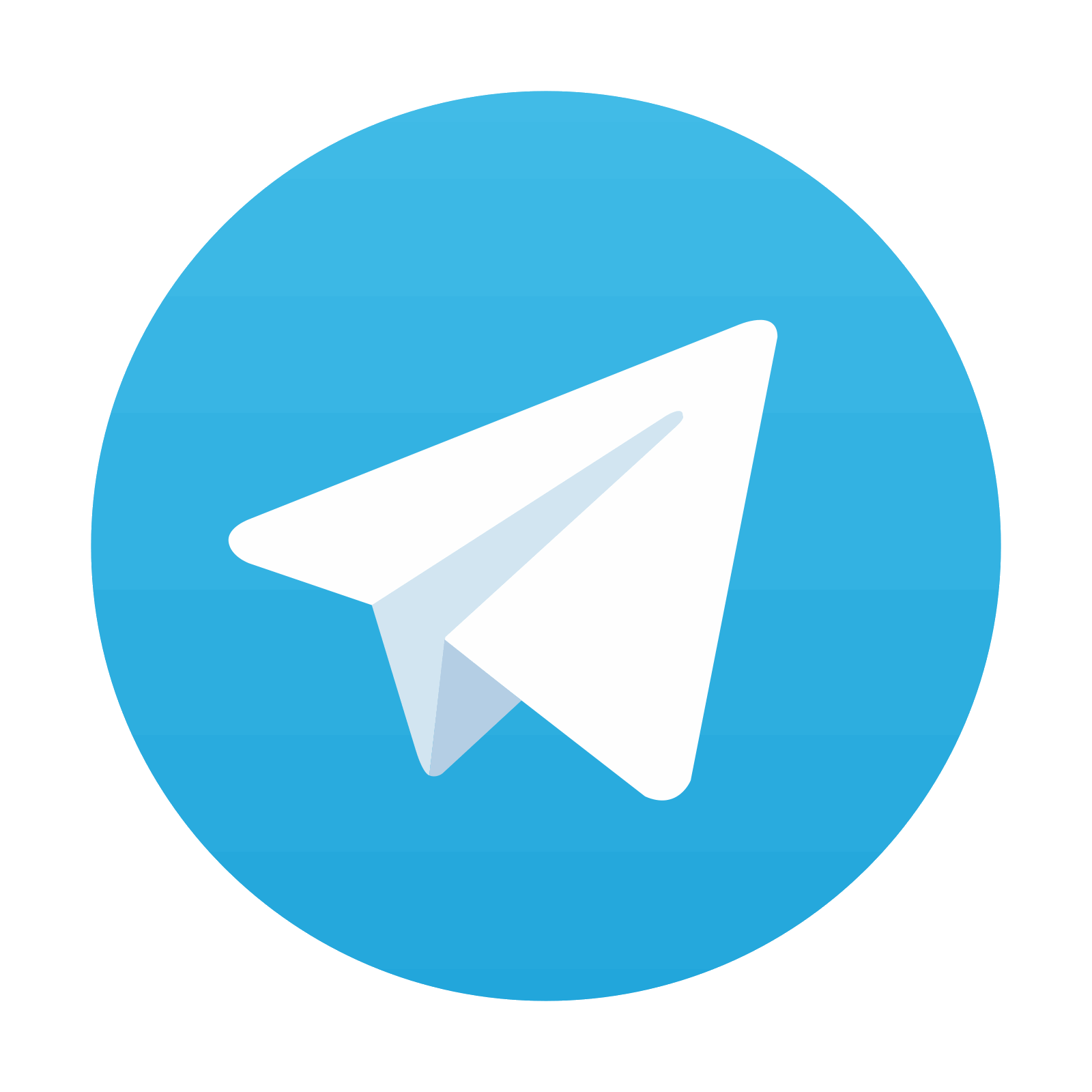
Stay updated, free articles. Join our Telegram channel
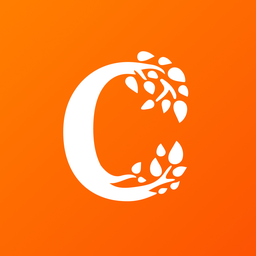
Full access? Get Clinical Tree
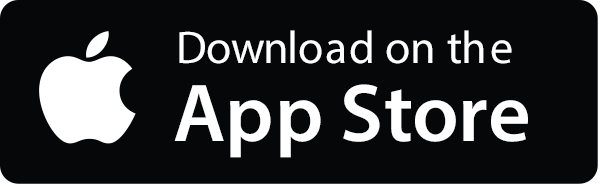
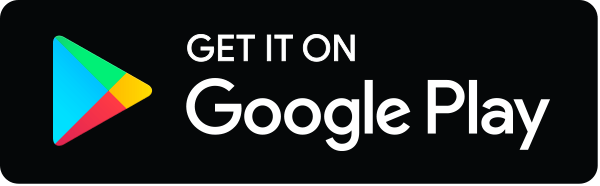