The first generation of cross-sectional brain imaging using computed tomography (CT), ultrasonography, and eventually MR imaging focused on determining structural or anatomic changes associated with brain disorders. The current state-of-the-art imaging, functional imaging, uses techniques such as CT and MR perfusion that allow determination of physiologic parameters in vivo. In parallel, tissue-based genomic, transcriptomic, and proteomic profiling of brain tumors has created several novel and exciting possibilities for molecular targeting of brain tumors. The next generation of imaging translates these molecular in vitro techniques to in vivo, noninvasive, targeted reconstruction of tumors and their microenvironments.
Key points
- •
The advent of genomic, proteomic, and high-throughput screening technologies has made available many new targets for brain tumor imaging; however, target availability and accessibility need to be carefully considered when designing imaging probes.
- •
Nanoparticles, although largely still only used in preclinical studies, are a versatile tool for targeted imaging of physiologic and molecular aspects of brain tumors through many clinically used modalities.
- •
Liposomes can be used to transport diverse payloads in vivo, including contrast agents and drugs, and may be functionalized to increase circulation half-life and achieve targeting specificity.
- •
Polymeric, gold, and iron oxide nanoparticles have been used for diverse applications in preclinical studies; however, the utility of other methods, such as quantum dots and self-assembling DNA molecules, is yet to be established.
Introduction
The development of nanoparticles involves manipulating a variety of molecular constructs, including metals, lipids, polymers, proteins, and nucleic acids. These particles typically range from 1 to 100 nm (although some may be as large as 400 nm) and may enhance imaging contrast through their intrinsic molecular properties or by serving as a scaffold for carrying imaging agents. Importantly, nanoparticles have been used therapeutically as well for targeted drug delivery and during surgical resection of tumors. Several approaches have been developed to functionalize nanoparticles to enable transport of water-soluble drugs, increase the half-life of the particles and their cargo in vivo, and minimize the side effect profile of toxic free agents.
This article provides an overview of the molecular targets available for brain tumor imaging, then focuses on the use of liposomal contrast agents for imaging some of these targets. It next discusses other classes of nanoparticles, including those that show potential in preclinical studies, such as gold nanoparticles, quantum dots, and self-assembling DNA molecules, and others that have been used in clinical studies, such as iron oxide nanoparticles.
Introduction
The development of nanoparticles involves manipulating a variety of molecular constructs, including metals, lipids, polymers, proteins, and nucleic acids. These particles typically range from 1 to 100 nm (although some may be as large as 400 nm) and may enhance imaging contrast through their intrinsic molecular properties or by serving as a scaffold for carrying imaging agents. Importantly, nanoparticles have been used therapeutically as well for targeted drug delivery and during surgical resection of tumors. Several approaches have been developed to functionalize nanoparticles to enable transport of water-soluble drugs, increase the half-life of the particles and their cargo in vivo, and minimize the side effect profile of toxic free agents.
This article provides an overview of the molecular targets available for brain tumor imaging, then focuses on the use of liposomal contrast agents for imaging some of these targets. It next discusses other classes of nanoparticles, including those that show potential in preclinical studies, such as gold nanoparticles, quantum dots, and self-assembling DNA molecules, and others that have been used in clinical studies, such as iron oxide nanoparticles.
Molecular targeting of brain tumors
The molecular properties and availability of cellular and molecular targets dramatically influences the type of imaging modality and the nanoparticle platform used for detection. The flow of detectable biological information is amplified by transcription and translation, starting with 2 copies of a gene in DNA, which is transcribed to 10 2 to 10 3 copies of messenger RNA (mRNA), which is then in turn translated into 10 2 to 10 6 polypeptides. These polypeptides may assemble to form enzymatic proteins, whose function can be further used to amplify signals from target detection. Several methods have been developed for the discovery of new targets and for the design of efficient, high-affinity probes for them. These approaches include genomic and transcriptomic sequencing to identify differentially expressed genes in brain tumors, high-throughput robotic screening, phage display, rational design, and combinatorial approaches.
Barriers to Nanoparticle and Probe Entry
Despite the rapid discovery of new targets and probes, the accessibility of cellular targets can limit their detection by nanoparticles because these molecules do not cross lipid bilayers and diffuse through cytoplasm easily ( Fig. 1 ). On entering the vasculature, the nanoparticles have to cross the endothelial layer to encounter the appropriate target cell. In the case of brain tumor targeting, the presence of the blood-brain barrier (BBB) presents an additional, significant challenge for the entry of nanoparticles. Once the nanoparticles gain entry into the brain parenchyma, the most accessible cellular targets are cell surface proteins. An additional barrier, the cell membrane, needs to be traversed for detection of most RNAs and cytosolic proteins. In contrast, both the cell and nuclear membranes must be crossed to reach DNA targets, making them the most difficult to detect. The localization of a particular target is therefore critical when considering the material properties and design of a nanoparticle.
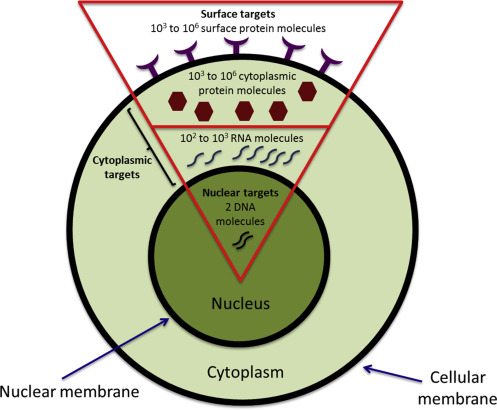
To circumvent the physiologic barriers that prevent nanoparticle and probe binding to their targets, several approaches have been used for brain tumor imaging. Surface chemical modifications are often used, such as polyethylene glycol (PEG) coating, and result in longer circulatory half-lives caused by decreased uptake by the reticuloendothelial system, which helps to achieve a uniform distribution of nanoparticles to increase distribution to all cellular targets. In certain circumstances, if the location of a tumor is known, localized delivery of nanoparticles may enhance detection of the desired target. Another approach allows cellular tracking through the detection of internalized nanoparticles into the cytoplasm from cell surface proteins. To gain entry into the brain, the use of ligand-bearing targeted nanoparticles for penetrating the BBB has also been extensively studied.
Signal Amplification
Given the difficulty of traversing cellular barriers and the low abundance of DNA and mRNA in cells, these are seldom targeted because significant signal amplification would be needed for appreciable detection. A few successful attempts have been made to tether nucleic acids to gold, carbon, and platinum nanoparticles. Several recent efforts have also enabled better detection of nucleic acid targets by exponential signal amplification. These approaches include nanosensors for specific DNA or RNA base pairing, rolling circle amplification, branched DNA amplification, hybridization chain reaction, and single-molecule RNA fluorescence in-situ hybridization. The applicability of these approaches to tumor imaging in clinical settings has yet to be established.
Unlike nucleic acids, proteins are abundant and accessible targets in living cells. As such, several approaches have been developed to amplify signal from protein detection. These approaches include manipulating the physiochemical behavior of a probe after target binding, harnessing unique cellular biochemistry to trap probes, and augmenting probe kinetics to increase effective target concentration.
Probe Classifications
Nanoparticles are in 3 classes of probes when used for brain tumor imaging: (1) compartment probes, (2) targeted probes, and (3) smart probes. Compartment probes are used to measure physiologic parameters, such as flow and perfusion. In this case, the properties of this probe force its compartmentalization to a specific region in the body, and allows an indirect measure of a particular process. Targeted probes contain a target-recognition moiety, which binds to the target molecule, and a contrast moiety, which is the signal being detected during imaging. An example of a targeted probe includes α v β 3 antibodies conjugated to gadolinium(III) [Gd(III)]–containing liposomes, which have been used for preclinical imaging of blood vessels. Smart probes require a trigger to activate, and in this way have an enhanced signal/background ratio compared with other probes. An example of a smart probe is EgadMe, a Gd chelating agent that occupies 7 of 8 Gd(III) coordination sites conjugated to a galactopyranose residue that blocks the remaining Gd(III) coordinate site. In the presence of a β-galactosidase, the blocked Gd(III) site is freed, allowing access to water for contrast enhancement. Calcium-activated and zinc-activated MR contrast agents have also been used in smart probes to detect activation of biochemical pathways in cells.
Liposomal contrast agents
Liposomes have been used as a particularly versatile class of nanoparticles for both diagnostic and therapeutic use in the central nervous system. They are composed either of a single bilayer, known as unilamellar vesicles, or multiple bilayers, known as multilamellar vesicles (MLVs). The approaches to fabricate liposomes and the ways in which they can be designed for targeting cellular and molecular processes for brain tumor imaging are discussed later.
Design and Functional Properties of Liposomes
Liposomes can vary in size depending on the method used to synthesize them. These methods include high-pressure extrusion and sonication. High-pressure extrusion is used for the synthesis of MLVs greater than 100 nm in diameter and large unilamellar vesicles (LUVs) 50 to 400 nm in diameter with remarkable consistency. Sonication is mostly used for the synthesis of small unilamellar vesicles (SUVs) of less than 30 to 50 nm.
The clearance of liposomes is predominantly mediated by the reticuloendothelial system, which consists of macrophages and other phagocytes in the hepatic and splenic compartments. This clearance depends on the size and surface properties of each liposome; traditional liposomes usually have half-lives of around minutes to hours, whereas liposomes larger than 200 nm have shorter half-lives because of more efficient immune clearance. These half-lives can be prolonged to more than 18 hours by coating the surface of liposomes with hydrophilic biopolymers such as PEG, creating so-called stealth liposomes that are better equipped to evade the immune system.
Liposomes have hydrophilic cores that may encapsulate imaging contrast agents or other compounds, such as drugs. As such, they have been used for diverse applications in both diagnostic imaging and therapeutics. Several trials have successfully shown the use of liposomes in delivering chemotherapeutic agents, including cytarabine, doxorubicin, and daunorubicin, as well as nucleic acids for gene therapy. They have further been used in image-guided therapeutic delivery by inclusion of both an imaging agent and a drug.
These nanoparticles have also been used for physiologic and molecular imaging using MR imaging and computed tomography (CT) by serving as carriers of gadolinium-based and iodine-based contrast agents. Liposomal contrast agents encapsulating a high payload of conventional iodine contrast agent molecules (∼1 million iodine atoms per liposome) have enabled ultrahigh-resolution CT imaging of rodent cerebrovasculature ( Fig. 2 ). Significant effort has been made to develop liposome-based MR imaging contrast agents. These nanoparticles have been successfully used as blood-pool contrast agents and for imaging neurovasculature and monitoring convection-enhanced drug delivery in vivo. As targeted probes, liposomes have been used to enhance targeting of brain tumors, for convection-enhanced delivery (CED), and to deliver boron for neutron-capture therapy.
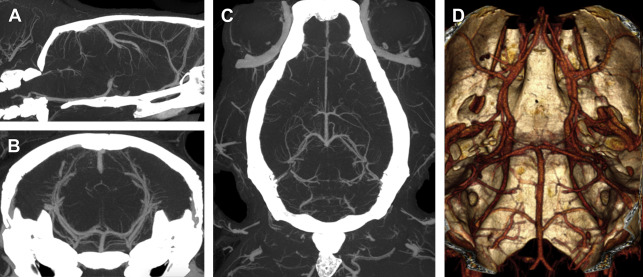
Targeting of Tumors Using Liposomes
Delivery of nanoparticles to tumors may involve passive diffusion or active targeting. The ability to create small liposome nanoparticles allows passive targeting of liposomes to brain tumors by means of diffusion through leaky vasculature surrounding tumor cells. Through this mechanism, referred to as the enhanced permeation and retention effect, liposomes carrying therapeutics or contrast agents can pool in the interstitial space of a tumor. The use of liposomes for molecular targeting of brain tumors involves conjugation of cell surface receptor recognizing antibodies to PEG chains on the liposome outer coat. Approaches to target multiple surface receptors using a single liposome have also been described. Liposomes can thus be targeted to the tumor microenvironment or to the endothelium of blood vessels within it. These approaches have been used to increase specificity of drug delivery by targeting the folate, transferrin, and epidermal growth factor receptors. In addition, triggered release liposomes have also been developed. These nanoparticles are designed to release contents, such as chemotherapeutics, in response to environmental changes such as temperature and pH.
Liposomes as MR Imaging–based Contrast Agents for Brain Tumors
The use of Gd core-encapsulated (CE-Gd) and surface-conjugated (SC-Gd) liposomes as T1-based MR imaging contrast agents has been validated extensively in animal models ( Fig. 3 ). Liposomes that use both core and surface Gd stores are referred to as dual-Gd liposomes. Of note, liposome cores encapsulated with ultrasmall superparamagnetic iron oxide particles (USPIOs) may be used for enhanced signal in T2-weighted MR imaging. These contrast agents have prolonged circulation, which provides uniform signal intensity and enhancement compared with traditional Gd chelates, thus making them ideal for steady-state imaging like MR angiography.
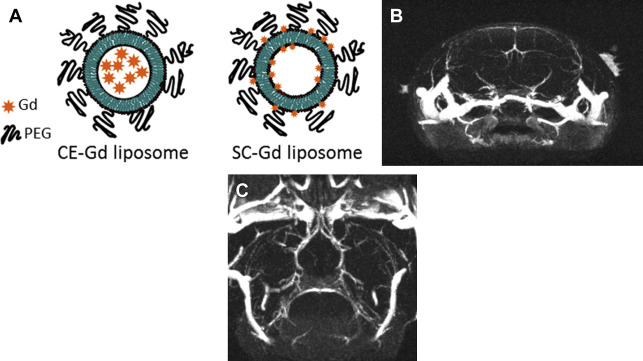
Thus, in preclinical studies, CE-Gd liposomes have been shown to result in lower background signal and increased vessel clarity in the spine and heart compared with conventional contrast agents. SC-Gd liposomes show higher T1 relaxivities compared with CE-Gd liposomes because water molecules do not have to diffuse through the lipid bilayer to interact with Gd atoms. As such, these agents have been used for higher-resolution imaging of both arterial and venous central nervous system vasculature, including the circle of Willis. Dual-Gd contrast agents afford the advantage of signal amplification from both modes of Gd pooling.
Unlike extracranial tumors, the presence of the BBB presents significant challenges to the entry of liposomes and other nanoparticle contrast agents into the brain. Focused ultrasonography-mediated enhancement of BBB permeability for augmenting intratumoral transport of liposomes has been studied. Active targeting of liposomes has also been examined for imaging of brain tumors. Importantly, although most nanoparticles are able to localize to brain tumors by extravasation through blood vessels, they are still large enough that this diffusion is limited by slow kinetics. Other smaller contrast agents are able to traverse the tumor microenvironment more rapidly through diffusion via brownian motion. CED is an approach whereby a burr hole is drilled into the brain, and a therapeutic or contrast agent is infused via a catheter directly into the tumor site. The positive pressure gradient of the infusion process reliably distributes the agent over the entire tumor volume, and, when the gradient normalizes, the distribution of the agent becomes diffusion limited. However, this approach has several limitations, including its invasive nature, limited versatility in terms of the shape and distribution of agent delivery, and often overtreatment, although overtreatment may be beneficial when trying to ensure tumor removal outside the expected margins.
Several preclinical studies have shown the safe and effective administration of liposome-encapsulated therapeutics to brain tumors using CED. These studies were limited in that they were unable to track the extent of nanoparticle delivery. Other studies in primate brains have used CE-Gd liposomes to monitor in real time the distribution of therapeutic nanoparticles infused into brain tumors by CED. The coencapsulation of both Gd contrast agents and therapeutics has enabled more accurate tracking of CED-mediated treatment of brain tumors.
Nonliposomal nanoparticles for brain tumor imaging
In addition to liposomes, several other types of nanoparticles have been used for brain tumor imaging, including other lipid-based nanoparticles, such as micelles, as well as polymeric, gold, and iron oxide nanoparticles. Recently, semiconductor nanocrystals, or quantum dots, that have tunable optical properties have also been used during surgery and in tumor imaging. A brief discussion of nucleic acid nanoparticles and their potential future application in molecular imaging is provided here.
Lipid-based Nanoparticles
Like liposomes, micelles and lipid-coated perfluorocarbon (PFC) nanoparticles are lipid-based nanoparticles. Micelles are approximately 10 to 50 nm in diameter. They are composed of several self-assembling amphiphilic molecules, which aggregate to form spherical particles that have a hydrophilic outer surface and a hydrophobic core. These nanoparticles can be used for imaging by tethering contrast moieties to the amphiphilic components. Often these micelles are PEGylated, which allows better immune evasion and prolonged circulation in the body. In addition, their surfaces can be further modified with proteins that facilitate cell-surface receptor binding in order to achieve tumor-specific targeting. An advantage of the hydrophobic core is that it may be used to deliver water-insoluble payloads.
PFC nanoparticles tend to be much larger than micelles, usually around 250 nm in diameter. They are composed of a lipid monolayer encapsulating a hydrophobic PFC core. The lipid monolayer can be used to carry contrast or therapeutic agents, or molecules to facilitate tumor targeting. PFC nanoparticles have been investigated preclinically for molecular imaging of brain tumors and the cardiovascular system. These nanoparticles may be used in both proton-based and 19 F-based MR imaging, because of the presence of fluorine atoms (perfluorocarbon) in the core interior. In addition to their use in MR imaging, PFCs along with porphysomes, which are liposomelike nanoparticles, have been used in photoacoustic imaging in the brain.
Polymeric Nanoparticles
Many years of investigation have gone into the synthesis and customization of biodegradable polymers, and this has been harnessed for molecular imaging. Dendrimers build the backbone of perhaps the most well-described class of polymeric nanoparticles. Dendrimers are highly branched, synthetic polymers that are effectively functionalized by conjugation to contrast moieties and may also be used for theraputics. These nanoparticles have been used effectively for brain tumor imaging with both MR imaging and CT. Importantly, antibody-conjugated dendrimers can be used for increased target specificity to brain tumors.
Biodegradable polymers including polylysine, polylactic acid, polylactic coglycolyic acid, and PEG-based polymers can be used to generate a homogeneous preparation of nanoparticles from 10 nm to 10 3 nm. These biopolymers can be effectively functionalized by fine-tuned control of nanoparticle size and structure, and by the conjugation of functional molecules or contrast agents. In particular, such polymeric nanoparticles have been used to effectively deliver chemotherapeutics to brain tumors.
Iron Oxide Nanoparticles
Perhaps the most widely described MR imaging contrast agents to date are iron oxide nanoparticles. These particles are composed of an iron oxide core that is stabilized with a coating of dextran or another hydrophilic polymer. They are developed either as USPIOs or supermagnetic iron oxide particles (SPIOs). USPIOs are less than 50 nm in diameter and have lower T2 relaxivities during MR imaging, thus they have been used as blood pool contrast agents. In contrast, SPIOs range between 50 and 200 nm in diameter, and have high T2 relaxivites. Therefore, they are used predominantly for T2-weighted MR imaging.
To date, these nanoparticles are the only ones approved for use as a contrast agent in humans, although several have been withdrawn by the manufacturers. They have a plasma half-life of more than 18-hours, which is significantly longer than traditional Gd-based contrast agents. They have been used as a contrast agent for brain tumor imaging and for visualizing blood vessels in the tumor microenvironment. When coated with dextran, these nanoparticles are rapidly taken up by T lymphocytes; as such, they can be effectively used to image the immune response in inflammatory diseases, tumors, and lymph nodes, such as for cancer staging. Given their long half-life and rapid uptake by dividing cells, these nanoparticles have been further used for MR imaging tracking of stem cells and microglia.
Gold Nanoparticles
A variety of gold nanoparticles have been developed and tested in preclinical imaging. The high attenuation of gold, compared with iodine, makes gold nanoparticles attractive for radiograph and CT imaging. Spectral CT imaging using a combination of gold nanoparticles and liposomal-iodine contrast agents has been investigated to simultaneously visualize tumor vasculature and intratumoral uptake of nanoparticles. Gold nanoparticles have a silica core covered by an extremely thin gold shell and have been investigated for multimodality imaging. These particles are extremely versatile because the core dimensions and shell thickness can be varied to impart the ability either absorb or scatter light, or x-rays. As a result, gold nanoparticles can be used in optical imaging and for highly sensitive surface-enhanced Raman scattering, which has been used for real-time visualization of tumor margins during surgical resection. Gold particles synthesized to absorb in the near-infrared spectrum have been used as a cancer therapeutic in certain animal models by means of photothermal ablation of cells. In humans, a similar approach has been used in trials for treatment of head and neck cancers. In addition, these nanoparticles are the most commonly used inorganic nanoparticle, along with quantum dots, in photoacoustic imaging.
Quantum Dots
As with gold nanoparticles, quantum dots have unique, flexible optical properties that can be modulated by altering their size. They are composed of a core made of a semiconductor material, usually a cadmium derivative, covered by an inert metallic layer. These nanoparticles have been used for optical imaging of the molecular properties of various tumors and may be helpful for guiding neurosurgical resection of brain tumors. Transferrin-conjugated quantum dots have been used for brain tumor targeting by virtue of binding to the transferrin receptor. The utility of these nanoparticles may be limited because they contain cadmium, which can be cytotoxic.
Self-assembling Nucleic Acid Nanoparticles
Recent advances in DNA origami have led to the development of self-assembling nucleic acid nanoparticles, which have the potential to be highly customizable in size and shape, and also able to carry a wide variety of payloads. Although still far away from practical use for clinical imaging, these nanoparticles have been used for small interfering RNA delivery to cells and may be conjugated to other nanoparticles, such as quantum dots and gold nanoparticles.
Summary
The promise of in vivo characterization of the attributes of brain tumors is becoming closer to reality each day. Although primarily in the preclinical realm, many of the technologies described in this article are being used in vivo. It is hoped that these technologies will continue to mature over the coming decade to more widespread clinical use in the same way as advanced functional approaches have come to fruition over the past decade.
Disclosure: The authors have nothing to disclose.
References
- 1. Davis K.R., Taveras J.M., New P.F., et al: Cerebral infarction diagnosis by computerized tomography. Analysis and evaluation of findings. Am J Roentgenol Radium Ther Nucl Med 1975; 124: pp. 643-660
- 2. Eastwood J.D., Lev M.H., Wintermark M., et al: Correlation of early dynamic CT perfusion imaging with whole-brain MR diffusion and perfusion imaging in acute hemispheric stroke. AJNR Am J Neuroradiol 2003; 24: pp. 1869-1875
- 3. Cancer Genome Atlas Research Network : Comprehensive genomic characterization defines human glioblastoma genes and core pathways. Nature 2008; 455: pp. 1061-1068
- 4. Geiger T., Cox J., Ostasiewicz P., et al: Super-SILAC mix for quantitative proteomics of human tumor tissue. Nat Methods 2010; 7: pp. 383-385
- 5. Parsons D.W., Jones S., Zhang X., et al: An integrated genomic analysis of human glioblastoma multiforme. Science 2008; 321: pp. 1807-1812
- 6. Pugh T.J., Weeraratne S.D., Archer T.C., et al: Medulloblastoma exome sequencing uncovers subtype-specific somatic mutations. Nature 2012; 488: pp. 106-110
- 7. Rausch T., Jones D.T., Zapatka M., et al: Genome sequencing of pediatric medulloblastoma links catastrophic DNA rearrangements with TP53 mutations. Cell 2012; 148: pp. 59-71
- 8. Cai W., and Chen X.: Multimodality molecular imaging of tumor angiogenesis. J Nucl Med 2008; 49: pp. 113s-128s
- 9. Gambhir S.S.: Molecular imaging of cancer with positron emission tomography. Nat Rev Cancer 2002; 2: pp. 683-693
- 10. Weissleder R.: Scaling down imaging: molecular mapping of cancer in mice. Nature reviews. Cancer 2002; 2: pp. 11-18
- 11. Weissleder R., Moore A., Mahmood U., et al: In vivo magnetic resonance imaging of transgene expression. Nat Med 2000; 6: pp. 351-355
- 12. Gao X., and Li C.: Nanoprobes visualizing gliomas by crossing the blood brain tumor barrier. Small 2014; 10: pp. 426-440
- 13. Leary S.P., Liu C.Y., Yu C., et al: Toward the emergence of nanoneurosurgery: part I–progress in nanoscience, nanotechnology, and the comprehension of events in the mesoscale realm. Neurosurgery 2005; 57: pp. 606-634
- 14. Zhang L., and Zhao D.: Applications of nanoparticles for brain cancer imaging and therapy. J Biomed Nanotechnol 2014; 10: pp. 1713-1731
- 15. Bertrand N., Wu J., Xu X., et al: Cancer nanotechnology: the impact of passive and active targeting in the era of modern cancer biology. Adv Drug Deliv Rev 2014; 66: pp. 2-25
- 16. Leary S.P., Liu C.Y., and Apuzzo M.L.: Toward the emergence of nanoneurosurgery: part II–nanomedicine: diagnostics and imaging at the nanoscale level. Neurosurgery 2006; 58: pp. 805-823
- 17. Barnaby S.N., Sita T.L., Petrosko S.H., et al: Therapeutic applications of spherical nucleic acids. Cancer Treat Res 2015; 166: pp. 23-50
- 18. Lee H., Lytton-Jean A.K., Chen Y., et al: Molecularly self-assembled nucleic acid nanoparticles for targeted in vivo siRNA delivery. Nat Nanotechnol 2012; 7: pp. 389-393
- 19. Lee T.J., Haque F., Vieweger M., et al: Functional assays for specific targeting and delivery of RNA nanoparticles to brain tumor. Methods Mol Biol 2015; 1297: pp. 137-152
- 20. Iv M., Telischak N., Feng D., et al: Clinical applications of iron oxide nanoparticles for magnetic resonance imaging of brain tumors. Nanomedicine (Lond) 2015; 10: pp. 993-1018
- 21. Kaluzova M., Bouras A., Machaidze R., et al: Targeted therapy of glioblastoma stem-like cells and tumor non-stem cells using cetuximab-conjugated iron-oxide nanoparticles. Oncotarget 2015; 6: pp. 8788-8806
- 22. Li J., Cai P., Shalviri A., et al: A multifunctional polymeric nanotheranostic system delivers doxorubicin and imaging agents across the blood-brain barrier targeting brain metastases of breast cancer. ACS Nano 2014; 8: pp. 9925-9940
- 23. Mehta A.G., Ghaghada K.B., and Mukundan S.: Future clinical applications of molecular imaging: nanoparticles, cellular probes, and imaging of gene expression. In Pillai J.J. (eds): Functional brain tumor imaging.. New York: Springer, 2014. pp. 225-237
- 24. Rudin M., and Weissleder R.: Molecular imaging in drug discovery and development. Nat Rev Drug Discov 2003; 2: pp. 123-131
- 25. Loi M., Di Paolo D., Soster M., et al: Novel phage display-derived neuroblastoma-targeting peptides potentiate the effect of drug nanocarriers in preclinical settings. J Control Release 2013; 170: pp. 233-241
- 26. Staquicini F.I., Sidman R.L., Arap W., et al: Phage display technology for stem cell delivery and systemic therapy. Adv Drug Deliv Rev 2010; 62: pp. 1213-1216
- 27. Toy R., Bauer L., Hoimes C., et al: Targeted nanotechnology for cancer imaging. Adv Drug Deliv Rev 2014; 76: pp. 79-97
- 28. Cheng Y., Morshed R.A., Auffinger B., et al: Multifunctional nanoparticles for brain tumor imaging and therapy. Adv Drug Deliv Rev 2014; 66: pp. 42-57
- 29. Doolittle N.D., Muldoon L.L., Culp A.Y., et al: Delivery of chemotherapeutics across the blood-brain barrier: challenges and advances. Adv Pharmacol 2014; 71: pp. 203-243
- 30. Meyers J.D., Doane T., Burda C., et al: Nanoparticles for imaging and treating brain cancer. Nanomedicine (Lond) 2013; 8: pp. 123-143
- 31. Suk J.S., Xu Q., Kim N., et al: PEGylation as a strategy for improving nanoparticle-based drug and gene delivery. Adv Drug Deliv Rev 2016; 99: pp. 28-51
- 32. Neuwelt E.A., Weissleder R., Nilaver G., et al: Delivery of virus-sized iron oxide particles to rodent CNS neurons. Neurosurgery 1994; 34: pp. 777-784
- 33. Lewin M., Carlesso N., Tung C.H., et al: Tat peptide-derivatized magnetic nanoparticles allow in vivo tracking and recovery of progenitor cells. Nat Biotechnol 2000; 18: pp. 410-414
- 34. Karathanasis E., and Ghaghada K.B.: Crossing the barrier: treatment of brain tumors using nanochain particles. Wiley Interdiscip Rev Nanomed Nanobiotechnol 2016; undefined:
- 35. van der Meel R., Vehmeijer L.J., Kok R.J., et al: Ligand-targeted particulate nanomedicines undergoing clinical evaluation: current status. Adv Drug Deliv Rev 2013; 65: pp. 1284-1298
- 36. Li H., Zhang Y., Wang L., et al: Nucleic acid detection using carbon nanoparticles as a fluorescent sensing platform. Chem Commun (Camb) 2011; 47: pp. 961-963
- 37. Polsky R., Gill R., Kaganovsky L., et al: Nucleic acid-functionalized Pt nanoparticles: catalytic labels for the amplified electrochemical detection of biomolecules. Anal Chem 2006; 78: pp. 2268-2271
- 38. Thaxton C.S., Georganopoulou D.G., and Mirkin C.A.: Gold nanoparticle probes for the detection of nucleic acid targets. Clin Chim Acta 2006; 363: pp. 120-126
- 39. Choi H.M., Chang J.Y., Trinh le A., et al: Programmable in situ amplification for multiplexed imaging of mRNA expression. Nat Biotechnol 2010; 28: pp. 1208-1212
- 40. Collins M.L., Irvine B., Tyner D., et al: A branched DNA signal amplification assay for quantification of nucleic acid targets below 100 molecules/ml. Nucleic Acids Res 1997; 25: pp. 2979-2984
- 41. Perez J.M., O’Loughin T., Simeone F.J., et al: DNA-based magnetic nanoparticle assembly acts as a magnetic relaxation nanoswitch allowing screening of DNA-cleaving agents. J Am Chem Soc 2002; 124: pp. 2856-2857
- 42. Raj A., van den Bogaard P., Rifkin S.A., et al: Imaging individual mRNA molecules using multiple singly labeled probes. Nat Methods 2008; 5: pp. 877-879
- 43. Smolina I.V., Demidov V.V., Cantor C.R., et al: Real-time monitoring of branched rolling-circle DNA amplification with peptide nucleic acid beacon. Anal Biochem 2004; 335: pp. 326-329
- 44. Barbet J., Peltier P., Bardet S., et al: Radioimmunodetection of medullary thyroid carcinoma using indium-111 bivalent hapten and anti-CEA x anti-DTPA-indium bispecific antibody. J Nucl Med 1998; 39: pp. 1172-1178
- 45. Gambhir S.S., Barrio J.R., Phelps M.E., et al: Imaging adenoviral-directed reporter gene expression in living animals with positron emission tomography. Proc Natl Acad Sci U S A 1999; 96: pp. 2333-2338
- 46. Hu S., Shively L., Raubitschek A., et al: Minibody: a novel engineered anti-carcinoembryonic antigen antibody fragment (single-chain Fv-CH3) which exhibits rapid, high-level targeting of xenografts. Cancer Res 1996; 56: pp. 3055-3061
- 47. Weissleder R., Tung C.H., Mahmood U., et al: In vivo imaging of tumors with protease-activated near-infrared fluorescent probes. Nat Biotechnol 1999; 17: pp. 375-378
- 48. Dzik-Jurasz A.S.: Molecular imaging in vivo: an introduction. Br J Radiol 2003; 76: pp. S98-S109
- 49. Schmieder A.H., Winter P.M., Williams T.A., et al: Molecular MR imaging of neovascular progression in the Vx2 tumor with alphavbeta3-targeted paramagnetic nanoparticles. Radiology 2013; 268: pp. 470-480
- 50. Louie A.Y., Hüber M.M., Ahrens E.T., et al: In vivo visualization of gene expression using magnetic resonance imaging. Nat Biotechnol 2000; 18: pp. 321-325
- 51. Li W.H., Parigi G., Fragai M., et al: Mechanistic studies of a calcium-dependent MRI contrast agent. Inorg Chem 2002; 41: pp. 4018-4024
- 52. Major J.L., Parigi G., Luchinat C., et al: The synthesis and in vitro testing of a zinc-activated MRI contrast agent. Proc Natl Acad Sci U S A 2007; 104: pp. 13881-13886
- 53. Szoka F., and Papahadjopoulos D.: Comparative properties and methods of preparation of lipid vesicles (liposomes). Annu Rev Biophys Bioeng 1980; 9: pp. 467-508
- 54. Yan X., Scherphof G.L., and Kamps J.A.: Liposome opsonization. J Liposome Res 2005; 15: pp. 109-139
- 55. Senior J.H.: Fate and behavior of liposomes in vivo: a review of controlling factors. Crit Rev Ther Drug Carrier Syst 1987; 3: pp. 123-193
- 56. Allen T.M., Hansen C., Martin F., et al: Liposomes containing synthetic lipid derivatives of poly(ethylene glycol) show prolonged circulation half-lives in vivo. Biochim Biophys Acta 1991; 1066: pp. 29-36
- 57. Klibanov A.L., Maruyama K., Torchilin V.P., et al: Amphipathic polyethyleneglycols effectively prolong the circulation time of liposomes. FEBS Lett 1990; 268: pp. 235-237
- 58. Woodle M.C., Newman M.S., and Cohen J.A.: Sterically stabilized liposomes: physical and biological properties. J Drug Target 1994; 2: pp. 397-403
- 59. Lassaletta A., Lopez-Ibor B., Mateos E., et al: Intrathecal liposomal cytarabine in children under 4 years with malignant brain tumors. J Neurooncol 2009; 95: pp. 65-69
- 60. Marina N.M., Cochrane D., Harney E., et al: Dose escalation and pharmacokinetics of pegylated liposomal doxorubicin (Doxil) in children with solid tumors: a pediatric oncology group study. Clin Cancer Res 2002; 8: pp. 413-418
- 61. Fabel K., Dietrich J., Hau P., et al: Long-term stabilization in patients with malignant glioma after treatment with liposomal doxorubicin. Cancer 2001; 92: pp. 1936-1942
- 62. Lippens R.J.: Liposomal daunorubicin (DaunoXome) in children with recurrent or progressive brain tumors. Pediatr Hematol Oncol 1999; 16: pp. 131-139
- 63. Yoshida J., and Mizuno M.: Clinical gene therapy for brain tumors. Liposomal delivery of anticancer molecule to glioma. J Neurooncol 2003; 65: pp. 261-267
- 64. Delac M., Motaln H., Ulrich H., et al: Aptamer for imaging and therapeutic targeting of brain tumor glioblastoma. Cytometry A 2015; 87: pp. 806-816
- 65. Karathanasis E., Chan L., Balusu S.R., et al: Multifunctional nanocarriers for mammographic quantification of tumor dosing and prognosis of breast cancer therapy. Biomaterials 2008; 29: pp. 4815-4822
- 66. Saito R., Bringas J.R., McKnight T.R., et al: Distribution of liposomes into brain and rat brain tumor models by convection-enhanced delivery monitored with magnetic resonance imaging. Cancer Res 2004; 64: pp. 2572-2579
- 67. Ghaghada K., Hawley C., Kawaji K., et al: T1 relaxivity of core-encapsulated gadolinium liposomal contrast agents–effect of liposome size and internal gadolinium concentration. Acad Radiol 2008; 15: pp. 1259-1263
- 68. Mukundan S., Ghaghada K.B., Badea C.T., et al: A liposomal nanoscale contrast agent for preclinical CT in mice. AJR Am J Roentgenol 2006; 186: pp. 300-307
- 69. Tilcock C., Unger E., Cullis P., et al: Liposomal Gd-DTPA: preparation and characterization of relaxivity. Radiology 1989; 171: pp. 77-80
- 70. Annapragada A.V., Hoffman E., Divekar A., et al: High-resolution CT vascular imaging using blood pool contrast agents. Methodist Debakey Cardiovasc J 2012; 8: pp. 18-22
- 71. Starosolski Z., Villamizar C.A., Rendon D., et al: Ultra high-resolution in vivo computed tomography imaging of mouse cerebrovasculature using a long circulating blood pool contrast agent. Sci Rep 2015; 5: pp. 10178
- 72. Howles G.P., Ghaghada K.B., Qi Y., et al: High-resolution magnetic resonance angiography in the mouse using a nanoparticle blood-pool contrast agent. Magn Reson Med 2009; 62: pp. 1447-1456
- 73. Krauze M.T., Forsayeth J., Park J.W., et al: Real-time imaging and quantification of brain delivery of liposomes. Pharm Res 2006; 23: pp. 2493-2504
- 74. Mulder W.J., Strijkers G.J., van Tilborg G.A., et al: Lipid-based nanoparticles for contrast-enhanced MRI and molecular imaging. NMR Biomed 2006; 19: pp. 142-164
- 75. Unger E.C., Shen D.K., and Fritz T.A.: Status of liposomes as MR contrast agents. J Magn Reson Imaging 1993; 3: pp. 195-198
- 76. Mulder W.J., Strijkers G.J., Griffioen A.W., et al: A liposomal system for contrast-enhanced magnetic resonance imaging of molecular targets. Bioconjug Chem 2004; 15: pp. 799-806
- 77. Mulder W.J., Strijkers G.J., Habets J.W., et al: MR molecular imaging and fluorescence microscopy for identification of activated tumor endothelium using a bimodal lipidic nanoparticle. FASEB J 2005; 19: pp. 2008-2010
- 78. Madhankumar A.B., Slagle-Webb B., Wang X., et al: Efficacy of interleukin-13 receptor-targeted liposomal doxorubicin in the intracranial brain tumor model. Mol Cancer Ther 2009; 8: pp. 648-654
- 79. Mamot C., Drummond D.C., Noble C.O., et al: Epidermal growth factor receptor-targeted immunoliposomes significantly enhance the efficacy of multiple anticancer drugs in vivo. Cancer Res 2005; 65: pp. 11631-11638
- 80. Grahn A.Y., Bankiewicz K.S., Dugich-Djordjevic M., et al: Non-PEGylated liposomes for convection-enhanced delivery of topotecan and gadodiamide in malignant glioma: initial experience. J Neurooncol 2009; 95: pp. 185-197
- 81. Krauze M.T., Nobel C.O., Kawaquchi T., et al: Convection-enhanced delivery of nanoliposomal CPT-11 (irinotecan) and PEGylated liposomal doxorubicin (Doxil) in rodent intracranial brain tumor xenografts. Neuro Oncol 2007; 9: pp. 393-403
- 82. Doi A., Kawabata S., Lida K., et al: Tumor-specific targeting of sodium borocaptate (BSH) to malignant glioma by transferrin-PEG liposomes: a modality for boron neutron capture therapy. J Neurooncol 2008; 87: pp. 287-294
- 83. Nakamura H.: Boron lipid-based liposomal boron delivery system for neutron capture therapy: recent development and future perspective. Future Med Chem 2013; 5: pp. 715-730
- 84. Ishida O., Maruyama K., Sasaki K., et al: Size-dependent extravasation and interstitial localization of polyethyleneglycol liposomes in solid tumor-bearing mice. Int J Pharm 1999; 190: pp. 49-56
- 85. McDonald D.M., and Choyke P.L.: Imaging of angiogenesis: from microscope to clinic. Nat Med 2003; 9: pp. 713-725
- 86. Alberts D.S., Muggia F.M., Carmichael J., et al: Efficacy and safety of liposomal anthracyclines in phase I/II clinical trials. Semin Oncol 2004; 31: pp. 53-90
- 87. Jain R.K.: Transport of molecules, particles, and cells in solid tumors. Annu Rev Biomed Eng 1999; 1: pp. 241-263
- 88. Jain R.K.: Delivery of molecular and cellular medicine to solid tumors. Adv Drug Deliv Rev 2012; 64: pp. 353-365
- 89. Maeda H.: Vascular permeability in cancer and infection as related to macromolecular drug delivery, with emphasis on the EPR effect for tumor-selective drug targeting. Proc Jpn Acad Ser B Phys Biol Sci 2012; 88: pp. 53-71
- 90. Madhankumar A.B., Slagle-Webb B., Mintz A., et al: Interleukin-13 receptor-targeted nanovesicles are a potential therapy for glioblastoma multiforme. Mol Cancer Ther 2006; 5: pp. 3162-3169
- 91. Saul J.M., Annapragada A.V., and Bellamkonda R.V.: A dual-ligand approach for enhancing targeting selectivity of therapeutic nanocarriers. J Control Release 2006; 114: pp. 277-287
- 92. Mamot C., Drummond D.C., Greiser U., et al: Epidermal growth factor receptor (EGFR)-targeted immunoliposomes mediate specific and efficient drug delivery to EGFR- and EGFRvIII-overexpressing tumor cells. Cancer Res 2003; 63: pp. 3154-3161
- 93. Kale A.A., and Torchilin V.P.: Environment-responsive multifunctional liposomes. Methods Mol Biol 2010; 605: pp. 213-242
- 94. Momekova D., Rangelov S., and Lambov N.: Long-circulating, pH-sensitive liposomes. Methods Mol Biol 2010; 605: pp. 527-544
- 95. Momekova D., Rangelov S., Yanev S., et al: Long-circulating, pH-sensitive liposomes sterically stabilized by copolymers bearing short blocks of lipid-mimetic units. Eur J Pharm Sci 2007; 32: pp. 308-317
- 96. Ponce A.M., Vujaskovic Z., Yuan F., et al: Hyperthermia mediated liposomal drug delivery. Int J Hyperthermia 2006; 22: pp. 205-213
- 97. Ghaghada K.B., Ravoori M., Sabapathy D., et al: New dual mode gadolinium nanoparticle contrast agent for magnetic resonance imaging. PLoS One 2009; 4: pp. e7628
- 98. Tilcock C., Ahknog Q.F., Koeing S.H., et al: The design of liposomal paramagnetic MR agents: effect of vesicle size upon the relaxivity of surface-incorporated lipophilic chelates. Magn Reson Med 1992; 27: pp. 44-51
- 99. Bulte J.W., and De Cuyper M.: Magnetoliposomes as contrast agents. Methods Enzymol 2003; 373: pp. 175-198
- 100. Ayyagari A.L., Zhang X., Ghaghada K.B., et al: Long-circulating liposomal contrast agents for magnetic resonance imaging. Magn Reson Med 2006; 55: pp. 1023-1029
- 101. Bucholz E., Ghaghada K., Qi Y., et al: Four-dimensional MR microscopy of the mouse heart using radial acquisition and liposomal gadolinium contrast agent. Magn Reson Med 2008; 60: pp. 111-118
- 102. Bucholz E., Ghaghada K., Qi Y., et al: Cardiovascular phenotyping of the mouse heart using a 4D radial acquisition and liposomal Gd-DTPA-BMA. Magn Reson Med 2010; 63: pp. 979-987
- 103. Ghaghada K.B., Bockhorst K.H., Mukundan S., et al: High-resolution vascular imaging of the rat spine using liposomal blood pool MR agent. AJNR Am J Neuroradiol 2007; 28: pp. 48-53
- 104. Aryal M., Park J., Vykhodtseva N., et al: Enhancement in blood-tumor barrier permeability and delivery of liposomal doxorubicin using focused ultrasound and microbubbles: evaluation during tumor progression in a rat glioma model. Phys Med Biol 2015; 60: pp. 2511-2527
- 105. Flament J., Geffroy F., Medina C., et al: In vivo CEST MR imaging of U87 mice brain tumor angiogenesis using targeted LipoCEST contrast agent at 7 T. Magn Reson Med 2013; 69: pp. 179-187
- 106. Liu X., Madhankumar A.B., Miller P.A., et al: MRI contrast agent for targeting glioma: interleukin-13 labeled liposome encapsulating gadolinium-DTPA. Neuro Oncol 2016; 18: pp. 691-699
- 107. Winter P.M., Pearce J., Chu Z., et al: Imaging of brain tumors with paramagnetic vesicles targeted to phosphatidylserine. J Magn Reson Imaging 2015; 41: pp. 1079-1087
- 108. Morrison P.F., Laske D.W., Bobo H., et al: High-flow microinfusion: tissue penetration and pharmacodynamics. Am J Physiol 1994; 266: pp. R292-R305
- 109. Ferguson S., and Lesniak M.S.: Convection enhanced drug delivery of novel therapeutic agents to malignant brain tumors. Curr Drug Deliv 2007; 4: pp. 169-180
- 110. Kaiser M.G., Parsa A.T., Fine R.L., et al: Tissue distribution and antitumor activity of topotecan delivered by intracerebral clysis in a rat glioma model. Neurosurgery 2000; 47: pp. 1391-1398
- 111. Saito R., Krauze M.T., Bringas J.R., et al: Gadolinium-loaded liposomes allow for real-time magnetic resonance imaging of convection-enhanced delivery in the primate brain. Exp Neurol 2005; 196: pp. 381-389
- 112. Dickinson P.J., LeCounter R.A., Higgins R.J., et al: Canine model of convection-enhanced delivery of liposomes containing CPT-11 monitored with real-time magnetic resonance imaging: laboratory investigation. J Neurosurg 2008; 108: pp. 989-998
- 113. Amirbekian V., Lipinski M.J., Briley-Saebo K.C., et al: Detecting and assessing macrophages in vivo to evaluate atherosclerosis noninvasively using molecular MRI. Proc Natl Acad Sci U S A 2007; 104: pp. 961-966
- 114. Dabholkar R.D., Sawant R.M., Mongayt D.A., et al: Polyethylene glycol-phosphatidylethanolamine conjugate (PEG-PE)-based mixed micelles: some properties, loading with paclitaxel, and modulation of P-glycoprotein-mediated efflux. Int J Pharm 2006; 315: pp. 148-157
- 115. Torchilin V.P.: Lipid-core micelles for targeted drug delivery. Curr Drug Deliv 2005; 2: pp. 319-327
- 116. Kaneda M.M., Caruthers S., Lanza G.M., et al: Perfluorocarbon nanoemulsions for quantitative molecular imaging and targeted therapeutics. Ann Biomed Eng 2009; 37: pp. 1922-1933
- 117. Tran T.D., Caruthers S.D., Huqhes M., et al: Clinical applications of perfluorocarbon nanoparticles for molecular imaging and targeted therapeutics. Int J Nanomedicine 2007; 2: pp. 515-526
- 118. Lanza G.M., Caruthers S.D., Hughes M., et al: 1H/19F magnetic resonance molecular imaging with perfluorocarbon nanoparticles. Curr Top Dev Biol 2005; 70: pp. 57-76
- 119. Wang D., Wu Y., and Xia J.: Review on photoacoustic imaging of the brain using nanoprobes. Neurophotonics 2016; 3: pp. 010901
- 120. Cheng C.J., Tietjen G.T., Saucier-Sawyer J.K., et al: A holistic approach to targeting disease with polymeric nanoparticles. Nat Rev Drug Discov 2015; 14: pp. 239-247
- 121. Tan M., Wu X., Jeong E.K., et al: Peptide-targeted nanoglobular Gd-DOTA monoamide conjugates for magnetic resonance cancer molecular imaging. Biomacromolecules 2010; 11: pp. 754-761
- 122. Wiener E.C., Brechbiel M.W., Brother H., et al: Dendrimer-based metal chelates: a new class of magnetic resonance imaging contrast agents. Magn Reson Med 1994; 31: pp. 1-8
- 123. Boswell C.A., Eck P.K., Regino C.A., et al: Synthesis, characterization, and biological evaluation of integrin alphavbeta3-targeted PAMAM dendrimers. Mol Pharm 2008; 5: pp. 527-539
- 124. Han L., Zhang A., Wang H., et al: Tat-BMPs-PAMAM conjugates enhance therapeutic effect of small interference RNA on U251 glioma cells in vitro and in vivo. Hum Gene Ther 2010; 21: pp. 417-426
- 125. Sarin H., Kanevsky A.S., Wu H., et al: Effective transvascular delivery of nanoparticles across the blood-brain tumor barrier into malignant glioma cells. J Transl Med 2008; 6: pp. 80
- 126. Singh P., Gupta U., Asthana A., et al: Folate and folate-PEG-PAMAM dendrimers: synthesis, characterization, and targeted anticancer drug delivery potential in tumor bearing mice. Bioconjug Chem 2008; 19: pp. 2239-2252
- 127. Yang W., Wu G., Barth R.F., et al: Molecular targeting and treatment of composite EGFR and EGFRvIII-positive gliomas using boronated monoclonal antibodies. Clin Cancer Res 2008; 14: pp. 883-891
- 128. Regino C.A., Walbridge S., Bernardo M., et al: A dual CT-MR dendrimer contrast agent as a surrogate marker for convection-enhanced delivery of intracerebral macromolecular therapeutic agents. Contrast Media Mol Imaging 2008; 3: pp. 2-8
- 129. Danson S., Ferry D., Alakhov V., et al: Phase I dose escalation and pharmacokinetic study of pluronic polymer-bound doxorubicin (SP1049C) in patients with advanced cancer. Br J Cancer 2004; 90: pp. 2085-2091
- 130. Kuroda J., Kuratsu J., Yasunaga M., et al: Antitumor effect of NK012, a 7-ethyl-10-hydroxycamptothecin-incorporating polymeric micelle, on U87MG orthotopic glioblastoma in mice compared with irinotecan hydrochloride in combination with bevacizumab. Clin Cancer Res 2010; 16: pp. 521-529
- 131. Torchilin V.P.: Polymeric contrast agents for medical imaging. Curr Pharm Biotechnol 2000; 1: pp. 183-215
- 132. Torchilin V.P.: PEG-based micelles as carriers of contrast agents for different imaging modalities. Adv Drug Deliv Rev 2002; 54: pp. 235-252
- 133. Inoue T., Yamashita Y., Nishihara M., et al: Therapeutic efficacy of a polymeric micellar doxorubicin infused by convection-enhanced delivery against intracranial 9L brain tumor models. Neurooncol 2009; 11: pp. 151-157
- 134. Weissleder R., Elizondo G., Wittenberg J., et al: Ultrasmall superparamagnetic iron oxide: characterization of a new class of contrast agents for MR imaging. Radiology 1990; 175: pp. 489-493
- 135. Laurent S., Boutry S., Mahieu I., et al: Iron oxide based MR contrast agents: from chemistry to cell labeling. Curr Med Chem 2009; 16: pp. 4712-4727
- 136. Laurent S., Forge D., Port M., et al: Magnetic iron oxide nanoparticles: synthesis, stabilization, vectorization, physicochemical characterizations, and biological applications. Chem Rev 2008; 108: pp. 2064-2110
- 137. Thorek D.L., Chen A.K., Czupryna J., et al: Superparamagnetic iron oxide nanoparticle probes for molecular imaging. Ann Biomed Eng 2006; 34: pp. 23-38
- 138. Li W., Tutton S., Vu A.T., et al: First-pass contrast-enhanced magnetic resonance angiography in humans using ferumoxytol, a novel ultrasmall superparamagnetic iron oxide (USPIO)-based blood pool agent. J Magn Reson Imaging 2005; 21: pp. 46-52
- 139. Tombach B., Reimer P., Bremer C., et al: First-pass and equilibrium-MRA of the aortoiliac region with a superparamagnetic iron oxide blood pool MR contrast agent (SH U 555 C): results of a human pilot study. NMR Biomed 2004; 17: pp. 500-506
- 140. Wang Y.X., Hussain S.M., and Krestin G.P.: Superparamagnetic iron oxide contrast agents: physicochemical characteristics and applications in MR imaging. Eur Radiol 2001; 11: pp. 2319-2331
- 141. Ros P.R., Freeny P.C., Harms S.E., et al: Hepatic MR imaging with ferumoxides: a multicenter clinical trial of the safety and efficacy in the detection of focal hepatic lesions. Radiology 1995; 196: pp. 481-488
- 142. Christoforidis G.A., Yang M., Kontzialis M.S., et al: High resolution ultra high field magnetic resonance imaging of glioma microvascularity and hypoxia using ultra-small particles of iron oxide. Invest Radiol 2009; 44: pp. 375-383
- 143. Gambarota G., and Leenders W.: Characterization of tumor vasculature in mouse brain by USPIO contrast-enhanced MRI. Methods Mol Biol 2011; 771: pp. 477-487
- 144. Neuwelt E.A., Várallayay C.G., Manninger S., et al: The potential of ferumoxytol nanoparticle magnetic resonance imaging, perfusion, and angiography in central nervous system malignancy: a pilot study. Neurosurgery 2007; 60: pp. 601-611
- 145. Varallyay C.G., Muldoon L.L., Gahramanov S., et al: Dynamic MRI using iron oxide nanoparticles to assess early vascular effects of antiangiogenic versus corticosteroid treatment in a glioma model. J Cereb Blood Flow Metab 2009; 29: pp. 853-860
- 146. Weinstein J.S., Varallyay C.G., Dosa E., et al: Superparamagnetic iron oxide nanoparticles: diagnostic magnetic resonance imaging and potential therapeutic applications in neurooncology and central nervous system inflammatory pathologies, a review. J Cereb Blood Flow Metab 2010; 30: pp. 15-35
- 147. Harisinghani M.G., Barentsz J., Hahn P.F., et al: Noninvasive detection of clinically occult lymph-node metastases in prostate cancer. N Engl J Med 2003; 348: pp. 2491-2499
- 148. Lahaye M.J., Engelen S.M., Kessels A.G., et al: USPIO-enhanced MR imaging for nodal staging in patients with primary rectal cancer: predictive criteria. Radiology 2008; 246: pp. 804-811
- 149. Fleige G., Nolte C., Synowitz M., et al: Magnetic labeling of activated microglia in experimental gliomas. Neoplasia 2001; 3: pp. 489-499
- 150. Thu M.S., Najbauer J., Kendall S.E., et al: Iron labeling and pre-clinical MRI visualization of therapeutic human neural stem cells in a murine glioma model. PLoS One 2009; 4: pp. e7218
- 151. Valable S., Barbier E.L., Bernaudin M., et al: In vivo MRI tracking of exogenous monocytes/macrophages targeting brain tumors in a rat model of glioma. Neuroimage 2008; 40: pp. 973-983
- 152. Wu X., Hu J., Zhou L., et al: In vivo tracking of superparamagnetic iron oxide nanoparticle-labeled mesenchymal stem cell tropism to malignant gliomas using magnetic resonance imaging. Laboratory investigation. J Neurosurg 2008; 108: pp. 320-329
- 153. Cole L.E., Ross R.D., Tilley J.M., et al: Gold nanoparticles as contrast agents in x-ray imaging and computed tomography. Nanomedicine (Lond) 2015; 10: pp. 321-341
- 154. Li W., and Chen X.: Gold nanoparticles for photoacoustic imaging. Nanomedicine (Lond) 2015; 10: pp. 299-320
- 155. Vo-Dinh T., Liu Y., Fales A.M., et al: SERS nanosensors and nanoreporters: golden opportunities in biomedical applications. Wiley Interdiscip Rev Nanomed Nanobiotechnol 2015; 7: pp. 17-33
- 156. Ashton J.R., West J.L., and Badea C.T.: In vivo small animal micro-CT using nanoparticle contrast agents. Front Pharmacol 2015; 6: pp. 256
- 157. Ashton J.R., Clark D.P., Moding E.J., et al: Dual-energy micro-CT functional imaging of primary lung cancer in mice using gold and iodine nanoparticle contrast agents: a validation study. PLoS One 2014; 9: pp. e88129
- 158. Clark D.P., Ghaghada K., Moding E.J., et al: In vivo characterization of tumor vasculature using iodine and gold nanoparticles and dual energy micro-CT. Phys Med Biol 2013; 58: pp. 1683-1704
- 159. Hirsch L.R., Gobin A.M., Lowery A.R., et al: Metal nanoshells. Ann Biomed Eng 2006; 34: pp. 15-22
- 160. Loo C., Hrisch L., Lee M.H., et al: Gold nanoshell bioconjugates for molecular imaging in living cells. Opt Lett 2005; 30: pp. 1012-1014
- 161. West J.L., and Halas N.J.: Engineered nanomaterials for biophotonics applications: improving sensing, imaging, and therapeutics. Annu Rev Biomed Eng 2003; 5: pp. 285-292
- 162. Lin A.W., Lewinski N.A., West J.L., et al: Optically tunable nanoparticle contrast agents for early cancer detection: model-based analysis of gold nanoshells. J Biomed Opt 2005; 10: pp. 064035
- 163. Talley C.E., Jackson J.B., Oubre C., et al: Surface-enhanced Raman scattering from individual au nanoparticles and nanoparticle dimer substrates. Nano Lett 2005; 5: pp. 1569-1574
- 164. Harmsen S., Huang R., Wall M.A., et al: Surface-enhanced resonance Raman scattering nanostars for high-precision cancer imaging. Sci Transl Med 2015; 7: pp. 271ra7
- 165. Hirsch L.R., Stafford R.J., Bankson J.A., et al: Nanoshell-mediated near-infrared thermal therapy of tumors under magnetic resonance guidance. Proc Natl Acad Sci U S A 2003; 100: pp. 13549-13554
- 166. Loo C., Lowery A., Halas N., et al: Immunotargeted nanoshells for integrated cancer imaging and therapy. Nano Lett 2005; 5: pp. 709-711
- 167. O’Neal D.P., Hirsch L.R., Halas N.J., et al: Photo-thermal tumor ablation in mice using near infrared-absorbing nanoparticles. Cancer Lett 2004; 209: pp. 171-176
- 168. Schwartz J.A., Shetty A.M., Price R.E., et al: Feasibility study of particle-assisted laser ablation of brain tumors in orthotopic canine model. Cancer Res 2009; 69: pp. 1659-1667
- 169. Reiss P., Protiere M., and Li L.: Core/shell semiconductor nanocrystals. Small 2009; 5: pp. 154-168
- 170. Jackson H., Muhammad O., Daneshvar H., et al: Quantum dots are phagocytized by macrophages and colocalize with experimental gliomas. Neurosurgery 2007; 60: pp. 524-529
- 171. Khalessi A.A., Liu C.Y., and Apuzzo M.L.: Neurosurgery and quantum dots: part I–state of the art. Neurosurgery 2009; 64: pp. 1015-1027
- 172. Popescu M.A., and Toms S.A.: In vivo optical imaging using quantum dots for the management of brain tumors. Expert Rev Mol Diagn 2006; 6: pp. 879-890
- 173. Smith A.M., Duan H., Mohs A.M., et al: Bioconjugated quantum dots for in vivo molecular and cellular imaging. Adv Drug Deliv Rev 2008; 60: pp. 1226-1240
- 174. Zhang Y., Lu F., Yager K.G., et al: A general strategy for the DNA-mediated self-assembly of functional nanoparticles into heterogeneous systems. Nat Nanotechnol 2013; 8: pp. 865-872
- 175. Chou L.Y., Zagorovsky K., and Chan W.C.: DNA assembly of nanoparticle superstructures for controlled biological delivery and elimination. Nat Nanotechnol 2014; 9: pp. 148-155
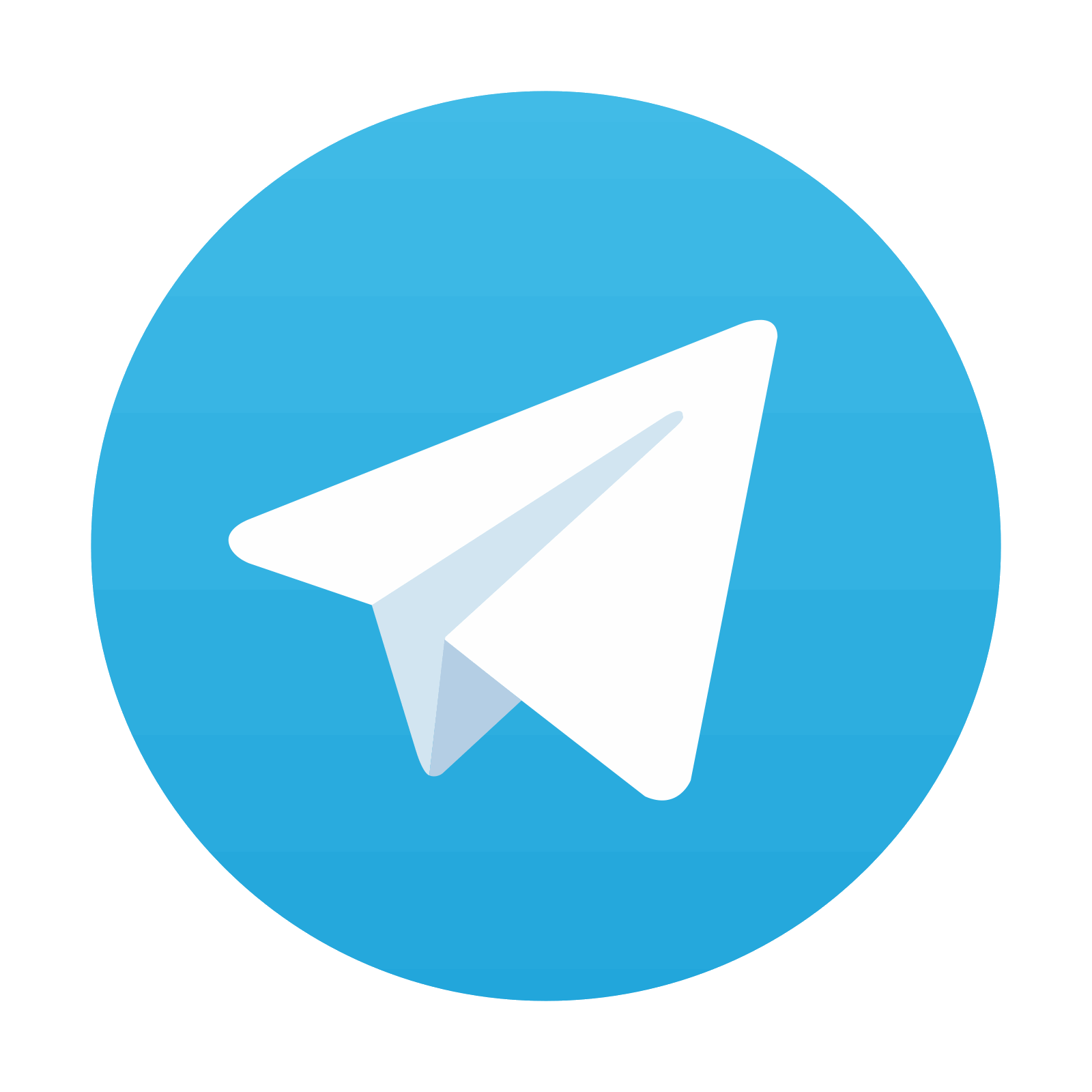
Stay updated, free articles. Join our Telegram channel
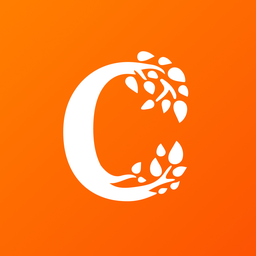
Full access? Get Clinical Tree
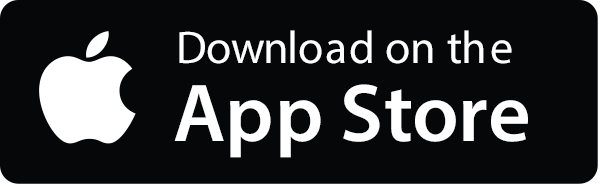
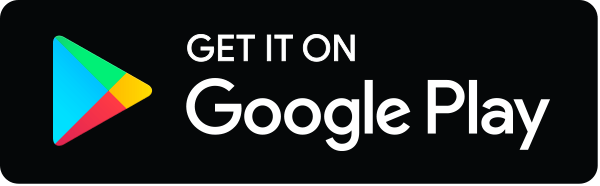