MR and CT Contrast Agents for Perfusion Imaging and Regulatory Issues
Henrik S. Thomsen
Peter Dawson
Michael F. Tweedle
Introduction
The term “contrast” has its origins in photography but is applicable to all imaging. Without contrast, there would be no image in any meaningful sense. In medical images there is some natural contrast that arises from the differing interactions between the different tissues, or between normal tissues and abnormalities arising within them, and the physical process being used to create the image, for example, X-rays (whether with plain films or computed tomography [CT]), magnetic resonance imaging (MRI), or ultrasound. To some extent, the natural contrast in the image may be improved by several maneuvers. In X-ray imaging the energy of the X-ray beam may be modified; in MRI a great variety of pulse sequences may be used with various values for basic parameters within each one.
In addition, artificial contrast-enhancing agents (CAs) have been developed for use in medical imaging. These are pharmaceutical preparations that may be administered, variously, by intra-arterial, intravenous, intra-articular, intracavity, intraoral, or per rectum routes. When injected or infused into the circulation they are carried to all perfused parts of the body where they modify the imaging process and resulting image. The various commercially available iodine-based CAs for X-ray and gadolinium (Gd)-based CAs for MRI are listed in Tables 7.1 and 7.3, respectively.
It is not difficult to see immediately, in general terms at least, how CAs may be used in dynamic imaging in a manner analogous to radioactive tracers in nuclear medicine techniques hence the field of functional imaging, including perfusion imaging.
Mechanisms of Action/Imaging Effects
Different physics and chemistry have to be exploited in the development of CAs used with X-rays and MRI. CAs used with X-ray techniques are very simple in principle, involving only the attachment of iodine atoms to low-toxicity molecules suitable for human administration. The use of X-ray CAs is in the thousands of tons annually. Iodine (I) is inexpensive and of a density detectable at millimolar concentrations in vivo, as it has a K-shell binding energy of some 33 kV and is an effective absorber of X-rays at energies above this level (and most effective at energies both above and close to it). This means that it is, like barium which is used traditionally in some preparations for gastrointestinal studies, a close-to-ideal element to utilize as an X-ray absorber. Every part of the body to which the iodine is carried will have enhanced X-ray absorption, and areas of different vascularity, and/or with different relative sizes of intravascular and extravascular spaces, will enhance to differing degrees. Abnormal areas (e.g., tumors) within a normal organ or tissue may enhance to a different extent than the surrounding normal tissue as a result of having different perfusion and tissue structure and may, therefore, stand out in greater contrast to the background normal tissue; that is, their conspicuity will also be enhanced in direct proportion to iodine concentration.
In MRI, on the other hand, in which the physics is much more complex than in X-ray imaging, a more subtle approach is used. MRIs are composites of volume elements, each of which has signal intensity dependent on the number of water protons and the T1 and T2 relaxation times of those protons. Here, paramagnetic (lanthanide/“rare earth” or “transition metal”) elements rather than iodine are attached to suitable carrier molecules or particles for human administration. The paramagnetic elements are catalysts that modify T1 and T2 relaxation times to an extent depending on the CA concentrations achieved locally as well as other environmental factors. This modification of relaxation times in turn modifies the image contrast. One group of CAs for MRI utilizes Gd firmly attached to one of various ligands. Such Gd chelates in solution exert their effects predominantly on T1 relaxation times. Another group of CAs utilizes iron (Fe) formulated as oxides into particles. Gadolinium is also a susceptibility agent. Hence, it has also a strong T2* effect. In addition, because of the microgradients produced by the susceptibility agent and proton diffusion in this gradient field, one can also see T2 effects. The particle suspensions exert their greatest effect on T2* relaxation times. The precise effect seen in resulting images in either case depends on several factors: field strength of the magnet, the imaging sequence adopted to emphasize T1 versus T2, the setting of basic parameters within the sequence, and so on. Generally, however, Gd-based agents show bright areas on T1-weighted scans, whereas FeO agents show black areas on T2-weighted scans.*
TABLE 7.1 STRUCTURES, TYPES, AND NAMES of COMMERCIAL IODINE-BASED X-RAY CONTRAST-ENHANCING AGENTS | |
---|---|
|
TABLE 7.2 PHYSICAL DATA FOR COMMERCIAL IODINATED X-RAY CONTRAST MEDIA | ||||||||||||||||||||||||||||||||||||||||||||||||||||||||||||||||||||||||||||||||||||||||||||||||||||||||||||||||||||||||||||||||||||||||||||||||||||||||||||||||||
---|---|---|---|---|---|---|---|---|---|---|---|---|---|---|---|---|---|---|---|---|---|---|---|---|---|---|---|---|---|---|---|---|---|---|---|---|---|---|---|---|---|---|---|---|---|---|---|---|---|---|---|---|---|---|---|---|---|---|---|---|---|---|---|---|---|---|---|---|---|---|---|---|---|---|---|---|---|---|---|---|---|---|---|---|---|---|---|---|---|---|---|---|---|---|---|---|---|---|---|---|---|---|---|---|---|---|---|---|---|---|---|---|---|---|---|---|---|---|---|---|---|---|---|---|---|---|---|---|---|---|---|---|---|---|---|---|---|---|---|---|---|---|---|---|---|---|---|---|---|---|---|---|---|---|---|---|---|---|---|---|---|---|
|
In the late 1970s organic nitroxides, paramagnetic transition metals, and other lanthanides were considered as T1 CA prototypes, Lauterbur creating the first ones from Mn2+. The characteristics that made Gd the best choice among alternatives are (a) its high magnetic moment, (b) its coordinated water lability, (c) the availability of strong chelating agents to solubilize it, and (d) Gd having only one available oxidation state. One oxidation state eliminated one potential form of toxicity due to redox reactions (moving electrons) in vivo. T1 catalysts (i.e., agents that accelerate T1 relaxation) need to operate on as many water molecules per second as possible; hence, labile (rapidly exchanging) metal–water coordination bonds are necessary, and Gd(III) was known to be quite labile. Although free Gd was obviously toxic owing to its immediate precipitation at pH 7, DTPA existed in the literature as 99mTc(DTPA) and Ln(DTPA) (Ln is a periodic table neighbor of Gd) nuclear agents that were known to be rapidly excreted and very stable. Finally, Gd3+, with its seven unpaired electrons in the seven 4f orbitals, yields the largest symmetrical paramagnetic moment. Unpaired electron spins create the desired high magnetic moment but also can participate negatively in the water proton relaxation if they are very short, reducing the proton T1 relaxation rate and increasing the T2 relaxation rate. The symmetric s-state of Gd3+ is the best electronic environment, (long electron relaxation times owing to inefficient pathways for the electrons to relax,1,†)to reduce T1 without excessive reduction of T2, yielding the optimum T1-weighted MRI scans. Gd therefore suggested itself early on as the element of choice as a contrast agent for MRI.2
Requirements for Contrast-Enhancing Agents
In addition to being able to achieve the desired effects on image contrast, a clinically viable CA must meet several other criteria: (a) it must be of low toxicity, (b) it must be chemically stable, (c) if metabolized to any degree its metabolic products must also be of low toxicity, and (d) it
must be wholly excreted from the body in a short time. While resident in the body it should ideally exert absolutely no physiologic effects whatsoever, unless by design. For perfusion imaging it requires the physical attributes necessary for administration of the drug as a bolus. Ideally, the CA is best administered as an aqueous solution in a small volume, making high solubility in water and high heat stability for heat sterilization very desirable. Unsurprisingly, no CA yet devised quite fulfills all these requirements perfectly, but the modern iodinated and Gd-chelate agents come remarkably close.
must be wholly excreted from the body in a short time. While resident in the body it should ideally exert absolutely no physiologic effects whatsoever, unless by design. For perfusion imaging it requires the physical attributes necessary for administration of the drug as a bolus. Ideally, the CA is best administered as an aqueous solution in a small volume, making high solubility in water and high heat stability for heat sterilization very desirable. Unsurprisingly, no CA yet devised quite fulfills all these requirements perfectly, but the modern iodinated and Gd-chelate agents come remarkably close.
TABLE 7.3 STRUCTURES, TYPES, NAMES, AND EXCRETION of COMMERCIAL Gd-BASED CONTRAST-ENHANCING AGENTS USED IN MRI | |
---|---|
|
Chemistry of Contrast-Enhancing Agents
The chemistry of contrast agents has evolved to optimally fulfill the physical requirements discussed previously. In
practice, this has led to commercialization of compounds of iodine for CT and other X-ray–based imaging, and compounds of Gd3+ for MRI imaging. Certain properties in common are the high concentrations of formulations (0.5 to 1 M) that necessitate structures with high water solubility (and therefore optimization of the resultant colligative properties [high osmolality and viscosity of formulations]), and the need for maximal acute and chronic tolerance (and therefore optimization of metabolism and excretion through the use of structures that do not interact with proteins and other biologic entities, except in a few cases by design).
practice, this has led to commercialization of compounds of iodine for CT and other X-ray–based imaging, and compounds of Gd3+ for MRI imaging. Certain properties in common are the high concentrations of formulations (0.5 to 1 M) that necessitate structures with high water solubility (and therefore optimization of the resultant colligative properties [high osmolality and viscosity of formulations]), and the need for maximal acute and chronic tolerance (and therefore optimization of metabolism and excretion through the use of structures that do not interact with proteins and other biologic entities, except in a few cases by design).
Iodinated X-Ray Contrast-Enhancing Agents
All modern X-ray CAs are 2,4,6-tri-iodinated benzene derivatives (Table 7.1). This structure evolved to provide maximum iodine as a percent of the weight of the molecule (up to ∼50%), with iodine bonded tightly to the carrier (>50 kcal bond). The one-, three-, and five-ring positions are occupied by moieties that (a) enhance the water solubility of the overall molecule and (b) sterically (with their bulk) protect the iodine atom and aromatic ring from interactions with proteins. Hundreds of molecules have been screened over nearly a century to arrive at the commercial structures available today.3 Commercial agents have water solubility from ∼0.5 to >1 M (up to 400 mg I/mL) and very high acute tolerance, measured by the administered dose that kills 50% of animals, LD50. Acute intravenous LD50 values for these agents in mice are greater than 50 mmol/kg (i.e., ∼50 times the human dose). They are highly stable owing to the greater than 50 kcal I-C (aromatic) bonds, allowing heat/autoclave sterilization of final solutions in sealed bottles, followed by shelf lives of 2 to 3 years. Terminal sterilization (namely, filling the vials, sealing them, then autoclaving at greater than 100°C) is the most efficient and effective method for pharmaceutical solutions. The osmolality and viscosity of their solutions are low enough in most cases to allow the extremely rapid bolus administrations required for perfusion studies. Finally, their biologic interaction is extraordinarily low with almost no protein binding, and thus chemo- and neurotolerance are high enough for intra-arterial and in some cases intrathecal administration. Nearly 100% of the agents are excreted unchanged in the urine.
Osmolality
Colligative properties are those that depend on the number of particles in solution, rather than their chemical properties. In the context of CAs, these are osmolality and viscosity. Osmolality of CAs will ideally match physiologic osmolality of ∼300 mOsm/kg water. Contrast agents remain exterior to cells. When the osmolality of the cell’s external environment is increased relative to its interior, water flows out of the cell and the cell crenates (shrinks). Table 7.1 shows monomers (one aromatic ring) and dimers (two aromatic rings). Historically, monomer molecules were invented in the 1920s with only two relatively exposed iodine atoms per molecule (iodopyracet) and moved through three iodine atoms per molecule (acetrizoate), partially protected with other groups, and then three iodine atoms per molecule with all six positions on the ring occupied, like diatrizoate. Diatrizoate and its ratio three cousins were solubilized by carboxylic acids, and therefore dissociated into positive and negative ions on dissolution in water so that each three-iodine molecule provided two particles in solution, making their iodine/particle ratio 3:2. Osmolality of the 1-M solutions was ∼2000 mOsm/kg water, their LD50 value (intravenously [IV] in mice) was ∼20 mmol/kg,4 and in human use they caused osmolality-related adverse reactions, such as vasodilation, cell crenation, and pain on injection. The modern monomeric “nonionic agents” (net zero overall charge), pioneered by Torsten Almén, substituted nonacidic hydroxyl groups for the carboxylic acids (see Table 7.1) and have therefore an improved iodine particle ratio of 3:1 and osmolalities of 1-M solutions of ∼700 to 900 mOsm/kg (Table 7.2). There are also dimeric forms of both ionic and nonionic CAs that start with two chemically connected aromatic rings solubilized by either a single carboxylic acid or hydroxyalkyl groups. (Notice, a dimer is a molecular complex consisting of two identical molecules linked together or, in this context, nearly identical.) Osmolality is the primary driver for commercializing the dimers, the ionic ones having osmolality similar to nonionic monomers, and the nonionic dimers finally achieving physiologic osmolality, albeit at no higher than 320 mg I/kg (∼0.7 M) because of excessive viscosity at higher concentrations that effectively prevents efficient administration by syringe. The nonionic CAs actually have osmolality below the theoretically expected numbers because the molecules tend to self-associate at high concentrations, reducing the number of particles in solution. The nonionic dimeric agents, of which there are now two commercial representatives, are still hypo-osmolar with respect to plasma at concentrations up to 320 mg I/mL and are therefore formulated with added salts to bring the osmolality to physiologic values. It is unlikely that self-association of CAs is maintained in vivo as they are quickly diluted in blood.
Hyperosmolar solutions are associated with several adverse effects: (a) they are irritants to endothelium; (b) they cause vasodilatation and sometimes uncomfortable flushing for patients; (c) they cause pain on arterial injection, particularly into smaller arteries; (d) they are associated with fluid shifts with hemodynamic consequences; and (e) they cause distortion and rigidification of cells in the circulation. The high-osmolality ionic agents, no longer in significant use in the Western world, were associated with considerable osmolality-related side effects, including excruciating pain
in some studies, including femoral run-off imaging. The “low osmolality,” nonionic monomeric agents are associated with only small osmotic effects, engendering little in the way of fluid shifts and other physiologic effects, and are very acceptable to patients. Nonionic dimers are theoretically superior in this regard, but the improvement is limited to the applications with intermediate concentrations (where the nonionic monomers are already relatively painless), and they are more expensive to produce.
in some studies, including femoral run-off imaging. The “low osmolality,” nonionic monomeric agents are associated with only small osmotic effects, engendering little in the way of fluid shifts and other physiologic effects, and are very acceptable to patients. Nonionic dimers are theoretically superior in this regard, but the improvement is limited to the applications with intermediate concentrations (where the nonionic monomers are already relatively painless), and they are more expensive to produce.
Viscosity
The viscosity of a formulation of CAs is an important practical matter for hand injection where a high viscosity requires more pressure to deliver through a syringe needle. This is becoming a lower-order problem, however, now that power injectors are almost invariably used in the most common application of iodinated agents, computed tomography. There are significant differences among viscosities of the various commercially available agents, the nonionic dimer being the most viscous of all (Table 7.2). Viscosities are a function of solution concentration, molecular shape, and weak interactions among the CAs and water molecules, including CA self-association. Theoretically, a perfectly spherical CA that did not interact with itself or water would have the lowest viscosity. The move from ionic to nonionic agents actually increased viscosity while decreasing osmolality and toxicity. The new dimers continue this trend to the point that 1 M (i.e., 370 to 400 mg I/mL) formulations are too viscous to use. Temperature affects viscosity strongly, and viscosity of the CAs may be markedly reduced by warming before injection to body temperature. The warming of contrast agents is also generally a best practice. CAs are usually stored at room temperature (∼18°C to 22°C), which is quite different from body temperature and may cause some discomfort to the patient during injection. There is speculation that both osmolality and viscosity can affect kidney toxicity (e.g., contrast-induced nephropathy [CIN]) because the CAs can be heavily concentrated in the collecting systems of the kidneys during their renal excretion.
Chemotoxicity
CAs are known also to possess “molecular toxicity”/“chemotoxicity,” a phenomenon thought to arise mostly from nonspecific and weak protein binding, which is in part determined by the relative hydrophilicity/hydrophobicity (partition coefficient) of the molecules and other unpredictable molecular characteristics. Quite separately from the hyperosmolality effects discussed earlier, this chemotoxicity of CAs may damage endothelium, distort cells in blood, and have inhibitory effects on enzymes. The chemotoxicities of the modern agents, and particularly of the nonionic dimeric agent, are much reduced in comparison with those of their predecessors (LD50 values IV in mice are two to three times greater in nonionic agents).5 This combination of low chemotoxicity and low (or, in the case of the dimer, iso-) osmolality renders the modern agents the closest approach yet achieved to a “physiologic” agent.6,7
GD Chelate Contrast-Enhancing Agents
Gd cannot be administered as simple inorganic solutions such as chlorides or sulphates because the simple salts of Gd hydrolyze (i.e., chemically react with water) immediately to form insoluble oxides and hydroxides at pH 7 that are retained for very long periods in macrophage organs like liver and bone. Mice LD50 values for injected raw Gd ions are about 0.1 to 0.3 mmol/kg, or about equal to a standard human dose of a current Gd-based CA, an unacceptable ratio of one (compared to the ≥50 in commercial X-ray and Gd MRI CAs).8 To be used as a CA, Gd must be firmly and stably bound to some carrier molecule that solubilizes it and prevents hydrolysis, while still allowing the catalysis of water proton relaxation, and in turn is rapidly excreted, carrying the heavy metal ion out of the body. The key to meeting these diverse requirements lies in the application of coordination chemistry, specifically chelation chemistry.9,10 In chemistry, a coordination complex or metal complex is an ion (Gd in our case) bound to a surrounding array of molecules or anions, which are in turn known as ligands. The word “chelation” is derived from the Greek χηλη′, chelè, meaning “claw,” because the carrier molecules, formally called chelating ligands or chelating agents, tend to surround the Gd atom like the claws of a lobster. “Chelation” is the formation of two or more separate, simultaneous bonds between a ligand and a single metal atom.
Certain polycarboxylic acids, such as DTPA (diethylene tetra-amine penta-acetate, Table 7.3), a prototypical ligand in this field, have been known to coordinate metal ions strongly since the 1950s. When two atoms from a single ligand molecule bind to a metal, they form a “chelate ring.” The chelate rings made up of five members (e.g., Gd-O-C-C-N or Gd-N-C-C-NN), found in the Gd CAs, are especially stable (much more so than four- or six-membered rings). A single ligand making two such rings with a metal ion is generally more than twice as strongly bound as one with only one ring, and the effect is highly nonlinear. Thus (see Table 7.5 and Keq equation later), chelating ligands with two Gd-O-C-C-NN rings in iminodiacetic acid (IDA) have binding constants (a measure of strength of binding) of 107 M−1, whereas Gd-EDTA (ethylene tretra-amine penta-acetate), which has four Gd-O-C-C-NN rings and one Gd-N-C-C-NN ring, has a binding constant of 1017 M−1, and Gd-DTPA, with seven rings, has a binding constant of 1022 M−1. As a measure of the bonding strength actually required by Gd-based CAs in vivo, Gd(EDTA)−, despite having a very high binding
constant, has an LD50 almost as low as free, unchelated Gd in Table 7.5.11 Gd(EDTA) precipitates on injection because the EDTA chelating agent is too weakly bound to Gd and allows Gd to be rapidly hydrolyzed and dechelated (removed from the EDTA chelate). Hence, the chelating ligands in the Gd CAs used commercially are truly remarkable chemical entities; they are among the strongest metal chelates known.12
constant, has an LD50 almost as low as free, unchelated Gd in Table 7.5.11 Gd(EDTA) precipitates on injection because the EDTA chelating agent is too weakly bound to Gd and allows Gd to be rapidly hydrolyzed and dechelated (removed from the EDTA chelate). Hence, the chelating ligands in the Gd CAs used commercially are truly remarkable chemical entities; they are among the strongest metal chelates known.12
![]() FIGURE 7.1. Relaxivity depends on a fast exchange of bulk and Gd-bound (coordinated) water molecules, and on the distance between the water protons and the Gd ion, to the sixth power, r6. (From Caravan P, Ellison JJ, McMurry TJ, et al. Gadolinium(III) chelates as MRI contrast agents: structure, dynamics, and applications. Chem Rev. 1999;99:2293–2352.) |
Gd(III) is a 3+ charged ion with nominally nine sites at which another chemical can bond to it. When the DTPA ligand is used, eight of these sites are occupied by the three N and five O− in the carboxyl groups. The ninth Gd site is available for fast exchange of water molecules between the “inner sphere” (bonded directly to Gd) and the bulk (nonbonded) water. This feature is necessary for strong water proton relaxation because (a) the Gd relaxation of the water proton depends inversely on r,6 where r is the distance between Gd and the water proton,10 so water protons need to be as close as possible to Gd, and (b) the time it takes Gd to relax a proton is about 1 million times faster than the 1-second MRI experiment, so the optimal effect on bulk water occurs when a million water molecules per second can bind Gd and exchange with another water molecule in the bulk water (i.e., rapid “exchange”)13 (Fig. 7.1). All commercial Gd chelates except protein binders achieve similar effects on proton relaxation and visibility in MRIs, each having a single water molecule bound and in rapid exchange equilibrium with the bulk water of their surroundings (Fig. 7.1). Obviously, a naked Gd ion will have up to nine bound water molecules and higher relaxivity than Gd chelates, but solubility and stability of the Gd in vivo necessitate the use of the chelates. Likewise, any polydentate ligand that used all of the Gd coordination sites in binding the ligand would all but eliminate its relaxation effectiveness. Protein binders are a special case. Experimental molecules exist with two water molecules exposed14,15 that are at least close to acceptable tolerance levels, but so far, molecules with more than one water exchanging† have not been proven in animals to be acceptable in stability or tolerance and remain untested in humans.
For Gd CAs the choice of ligand structure influences the compound’s chemical and biologic stability and the colligative properties of its formulations, both of which in turn influence tolerance, pharmacokinetics, clinical uses, and contraindications. Two fundamental structural types are in use: linear seven-ring structures derived from DTPA, and macrocyclic eight-ring structures derived from DOTA (dodecane tetra-acetic acid). The fundamental difference in these structures is that the chain of nitrogen and carbon atoms making up the backbone of the chelating agent is open on both ends in linear agents and closed into a single loop in macrocycles (Table 7.3). As in the case of X-ray CAs, elaboration of these basic structures can produce both ionic and nonionic molecules, and further derivatization has also produced molecules with variable excretion patterns and selective protein binding.
Examination of Gd(DTPA)2− (Table 7.3) reveals that the complex carries charges +3 from the Gd3+ ion and −5 from the five COOH groups. The latter must ionize and deprotonate to COO− to bind to the Gd3+ ion. The net charge when DTPA5− is fully coordinated to Gd3+ is therefore −2, and the Gd(DTPA)2− is formally written as a 2− anion, whose structure is in Table 7.3. Its generic name, the formal name of the active pharmaceutical agent, is gadopentetate. In medical literature, this compound is often abbreviated as Gd DTPA, Gd-DTPA, or Gd(DTPA), and other Gd CAs similarly. The Gd(DTPA)2− ion must be balanced in solution by two positive charges (cations). N-methylglucamine (meglumine, a sugar amine, abbreviated NMG+ in Table 7.3) is used for this purpose in the formulation. Other ionic Gd CAs use Na+ instead of NMG+. The molecular formula of the solid NMG salt of Gd(DTPA)2− is thus NMG2[Gd(DTPA)], formally, but in solution the two NMG+ ions are fully dissociated [unattached and independent of Gd(DTPA)] and the Gd(DTPA)2− ion is the active pharmaceutical entity. The agent is therefore ionic and each active molecule produces three particles in solution (all agents are dissolved in water) to contribute to osmolality: one Gd(DTPA)2− and two NMG+ ions. Similar reasoning explains the Gd(DOTA)− ion: four COO plus one Gd3+, making Gd(DOTA)− and Na[Gd(DOTA)] an ionic agent, gadoterate, with only two particles [one Gd(DOTA)− and one Na+] in solution for each active molecule, and thus lower osmolality of its formulations.
Osmolality
As with the iodinated agents, one way to reduce osmolality, without a reduction in concentration of the active agent, is to make a nonionic Gd(Ligand) molecule. Table 7.3 shows variants of the DTPA and DOTA structures in which there are only three negative groups (three COO− groups), the other two having been replaced by nonionizing methylamide or hydroxyalkyl groups. Thus, in Gd(DTPA-BMA) (gadodiamide), where the BMA stands for bis-methylamide, there are two nonionizing methyl amide groups. In this compound, the key idea is that the two oxygen atoms of the methylamide groups still bind to the Gd atom, and the three negative groups of the three remaining carboxyl (COO−) groups balance the three positive charges
of the Gd3+, producing a (charge) neutral molecule. Gd(DTPA-BMA) still contains seven chelate rings, but the molecule is charge neutral and does not need NMG or Na to balance it. The hydroxyalkyl replaces one COO− in DOTA to make Gd(HP-DO3A), an eight-ring chelate macrocycle. These are nonionic agents, by definition, and the structural unit provides only one particle in solution, the whole Gd(Ligand) molecule, which is the active pharmaceutical. With no Na+ or NMG+ counterions in solution, the reduction in osmolality realized is about a factor of 3 versus Gd(DTPA) and 2 versus Gd(DOTA) when the formulation concentration is equal at 0.5 M. The osmolalities of these materials are shown in Table 7.4. The osmolality of the 0.5-M Gd CA formulation of the simple DTPA compound is about the same as that of a conventional ionic iodinated agent at ≈370 mg I/mL concentration, whereas the osmolalities of the two nonionic Gd CAs are far lower. The nonionics followed quickly after the launch of the ionic molecules, so that by 1992, Gd(DTPA)2−, Gd(DTPA-BMA), Gd(DOTA)−, and Gd(HP-DO3A) were each marketed worldwide, although Gd(DOTA)− commercialization was and is still limited compared to the other three.
of the Gd3+, producing a (charge) neutral molecule. Gd(DTPA-BMA) still contains seven chelate rings, but the molecule is charge neutral and does not need NMG or Na to balance it. The hydroxyalkyl replaces one COO− in DOTA to make Gd(HP-DO3A), an eight-ring chelate macrocycle. These are nonionic agents, by definition, and the structural unit provides only one particle in solution, the whole Gd(Ligand) molecule, which is the active pharmaceutical. With no Na+ or NMG+ counterions in solution, the reduction in osmolality realized is about a factor of 3 versus Gd(DTPA) and 2 versus Gd(DOTA) when the formulation concentration is equal at 0.5 M. The osmolalities of these materials are shown in Table 7.4. The osmolality of the 0.5-M Gd CA formulation of the simple DTPA compound is about the same as that of a conventional ionic iodinated agent at ≈370 mg I/mL concentration, whereas the osmolalities of the two nonionic Gd CAs are far lower. The nonionics followed quickly after the launch of the ionic molecules, so that by 1992, Gd(DTPA)2−, Gd(DTPA-BMA), Gd(DOTA)−, and Gd(HP-DO3A) were each marketed worldwide, although Gd(DOTA)− commercialization was and is still limited compared to the other three.
Although nonionic X-ray agents showed a vast improvement in tolerance over their ionic predecessors, the nonionic MRI agent improvements were more modest. The primary reason for this is that the dose of Gd CA is only about 10% of the dose of X-ray CAs. The ionic X-ray CA volumes, as high as 200 mL of osmolality greater than 2000 mOsm/kg, were significant enough to cause systemic reactions,5 including blood pressure drops, whereas the 14 mL at ∼2000 mOsm/kg doses of ionic Gd CAs did not significantly affect systemic fluid shifts. As Gd CAs began to be administered at higher doses, the ionic linear agents were limited to 0.2 mmol/kg (i.e., double dose), whereas the nonionic Gd CAs can be administered at up to 0.3 mmol/kg (i.e., triple dose). In addition, with power injection, it became clear that a missed vein and consequent extravasation of Gd CAs could result in significant tissue necrosis and even limb loss with the highest-osmolality agent. There is a theoretical but unproven possible role for the effect of local, and brief, high osmolality, but if this was an important mechanism, nonionic Gd CAs would be expected to have fewer adverse reactions of an allergic or anaphylactoid nature, which they do not have.
TABLE 7.4 PHYSICAL DATA FOR COMMERCIAL Gd-BASED MRI CONTRAST MEDIA AT 37°C (RELAXIVITY AND STABILITY DATA FROM HAO D ET AL.16) | ||||||||||||||||||||||||||||||||||||||||||||||||||||||||||||||||||||||||||||||||||||||||||||||||||||||||||||||||||||||||||||||||||||||||||||||||||||||||||||||||||||||||||
---|---|---|---|---|---|---|---|---|---|---|---|---|---|---|---|---|---|---|---|---|---|---|---|---|---|---|---|---|---|---|---|---|---|---|---|---|---|---|---|---|---|---|---|---|---|---|---|---|---|---|---|---|---|---|---|---|---|---|---|---|---|---|---|---|---|---|---|---|---|---|---|---|---|---|---|---|---|---|---|---|---|---|---|---|---|---|---|---|---|---|---|---|---|---|---|---|---|---|---|---|---|---|---|---|---|---|---|---|---|---|---|---|---|---|---|---|---|---|---|---|---|---|---|---|---|---|---|---|---|---|---|---|---|---|---|---|---|---|---|---|---|---|---|---|---|---|---|---|---|---|---|---|---|---|---|---|---|---|---|---|---|---|---|---|---|---|---|---|---|---|
|
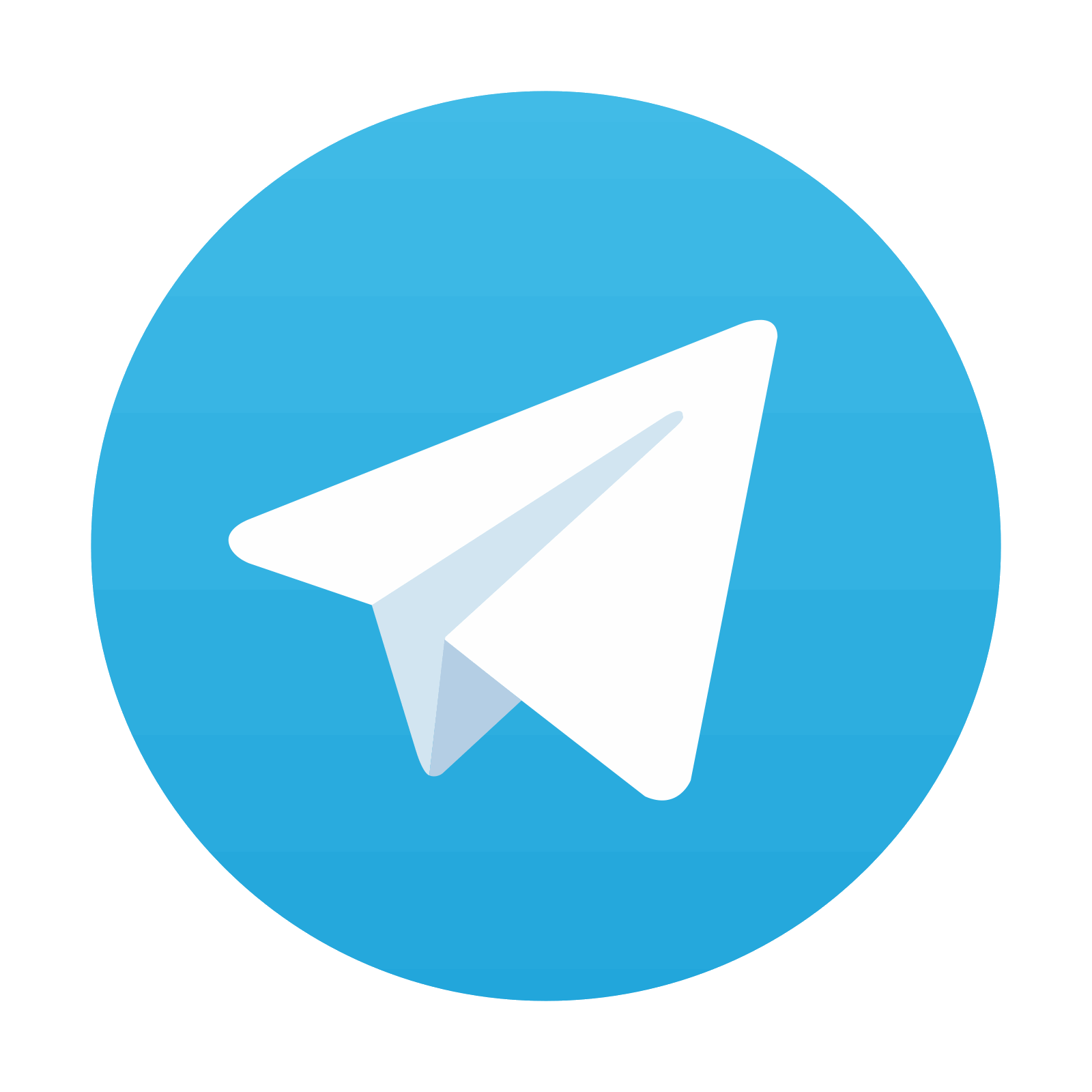
Stay updated, free articles. Join our Telegram channel
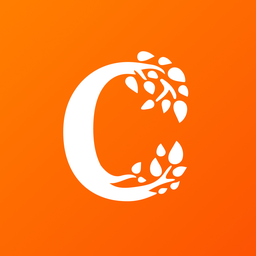
Full access? Get Clinical Tree
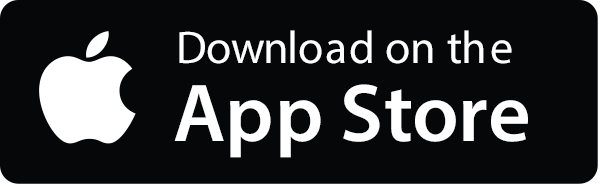
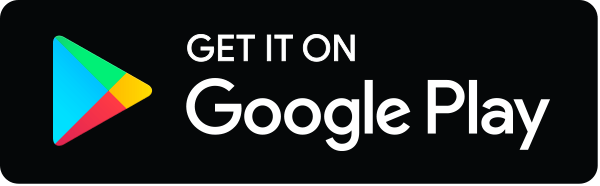