Fig. 2.1
Flow-void effect in a SE sequence. The moving blood protons that have already passed through the Field of View (FoV) are not subjected to the 180° refocusing impulse and do not provide a signal at the time the echo is received
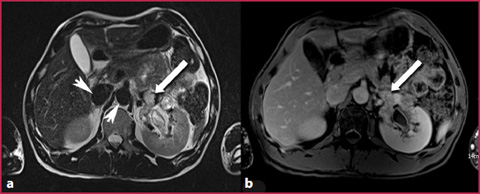
Fig. 2.2
a T2W TSE sequence: thrombosis involving the main renal vein and extending through the segmental left branches in which the normal flow-void effect is not visible (arrow), contrary to what is evident in the aorta and vena cava (arrowheads). b T1W sequence obtained after CM administration: the thrombus is clearly visible as a filling defect in the renal vein (arrow)
Table 2.1
Effect of variations in slice thickness on flow-void and in-flow phenomena
Sequences | Slice thickness | |
Flow-void | T2W SE | > if high |
In-flow | T1W GRE | > if low |
In-Flow Effect
The in–flow effect is observed especially in gradient-echo sequences and is the physical principle used in MRA Time–of–Flight sequences (ToF). When the stationary tissue protons are subjected to continuous RF pulses applied at extremely short repetition times (RT) (RT <T1 of the stationary tissues), the signal derived from them will be zero, because of constant saturation of their longitudinal magnetization. The moving blood protons, entering the acquisition volume, along a perpendicular direction (the angle between the vessel and the acquisition layer should be as close as possible to 90°) and crossing it at a constant and high speed, will not be subjected to longitudinal magnetization saturation due to the continuous RF pulses, and therefore will release a high signal at the time of echo reception, determining vascular enhancement related to in-flow effect (Figs. 2.3, 2.4). It is physically possible that this phenomenon occurs even in the spin-echo T1-weighted sequences, although its magnitude is reduced by coexistence with the flow-void effect. The in-flow degree increases with the decreasing of slice thickness, and vice versa (Table 2.1).
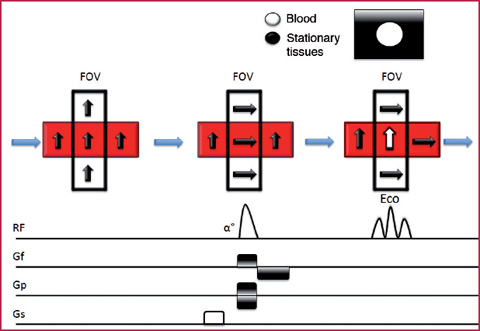
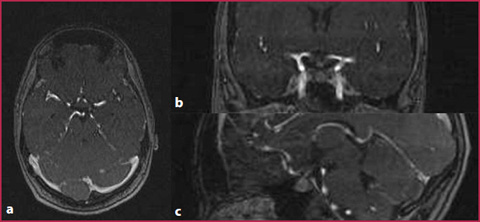
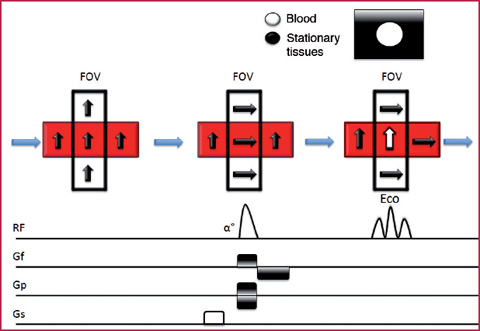
Fig. 2.3
In-flow effect in a GRE sequence. The protons of the stationary tissue are saturated by the continuous RF pulses applied at very short RT. The blood protons in the slice under examination do not suffer from the RF saturation effects and are able to release signals at the time the echo is received
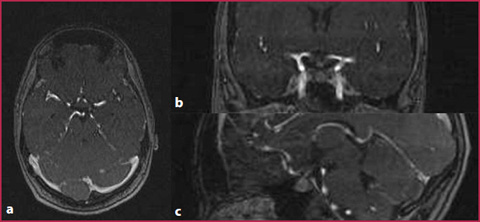
Fig. 2.4
MRA of the intracranial circulation obtained with the ToF technique, in which the in-flow effect is evident, without the use of a contrast medium. a Native axial acquisition. b Coronal plane reformation. c Sagittal plane reformation
Phase Shift Effect
The phase shift effect is observed when two consecutive magnetic gradients of equal intensity and amplitude but with opposite signals are applied to a volume under analysis and placed in a static magnetic field, causing a modification of the magnetization transverse vector. The vector intensity will be zero for the stationary tissue protons, but will present a value > 0 for substances in movement, such as the blood. The phase difference, and thus the fluid signal intensity, will be directly proportional to the flow speed along the direction of the gradient (Fig.2.5). The phase-shift effect is the physical phenomenon at the base of the Phase Contrast or PC sequences (Fig.2.6).
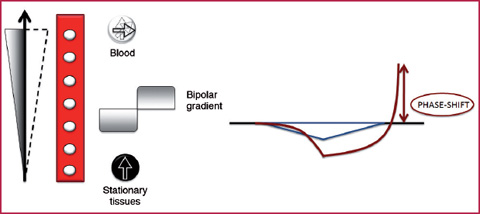
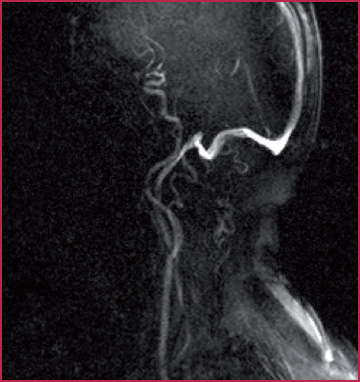
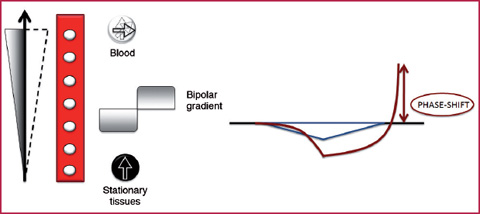
Fig. 2.5
Schematic representation of the phase-shift effect. The application of two magnetic gradients of equal intensity and amplitude and with a direction opposite to the blood in movement, determines a modification of the transverse magnetization in the moving spins which is different from zero and proportional to the flow velocity along the gradient direction
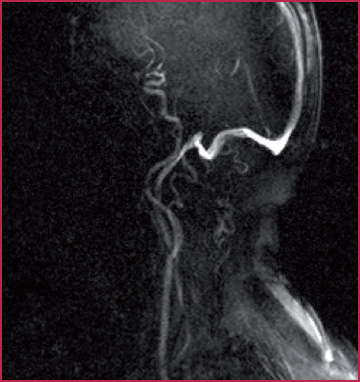
Fig. 2.6
MRA of the carotid arteries obtained with PC technique without the use of CM
Image Acquisition Techniques
Considering both the use of the flow phenomena previously described and of gadolinium contrast media (Gd CM), MRA techniques can be divided into two groups:
without the use of contrast media; this type of technique is based on the blood signal characteristics and can be divided into:
dark–blood techniques (or black blood), in which the intravascular signal will be lower than that of the surrounding stationary tissues;
bright–blood techniques (or white blood), in which the intravascular signal will be greater than that of the surrounding stationary tissues.
with contrast medium administration (Contrast Enhanced Magnetic Resonance Angiography, CE-MRA).
Non-Contrast MRA Techniques
This type of sequence is mainly used for the study of the myocardium, the vessel walls (Fig.2.7) and tight stenotic lesions associated with thrombotic disease. They are based on T1-weighted acquisitions in which the reduction of the intraluminal signal is obtained by the combined use of the following features:
Dark-Blood Sequences
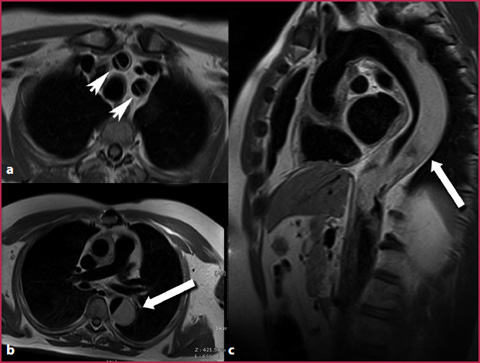
Short TR: the signal of the blood is saturated due to its long T1;
Medium TE (20–30 sec): in this way the protons in movement inside the voxel under examination are dephased; however, at the same time, the signal intensity of stationary tissues can be reduced, possibly resulting in an increase in motion artifacts;
Inversion Recovery (IR): use of preparation pulses with an inversion time chosen in order to reduce the signal intensity of the incoming blood;
Spatial Saturation: signal saturation of the blood outside the volume under examination, by means of the combined use of selective excitation pulses and subsequently dephasing gradients applied to decrease the protons’ transverse magnetization;
90-180° wash-out: this is obtained in the spin-echo sequences when the blood leaves the voxel under analysis in the period of time included between the application of the 90° RF pulse and at 180° so that the blood protons, not undergoing a refocusing impulse, will not produce any signal;
Gradient dephasing: this increases the movement-induced dephasement of the blood protons inside the voxels under analysis, for example by avoiding the use of techniques that cancel out the gradient moment.
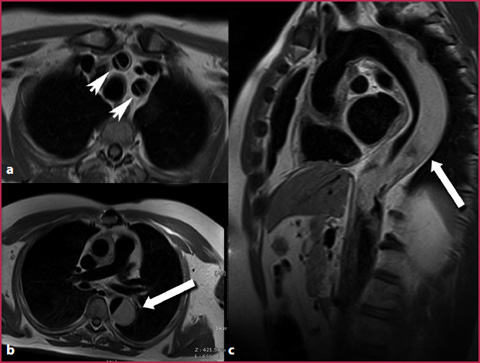
Fig. 2.7
T1W IR SE sequence obtained with the dark-blood technique in a patient with a type I aortic dissection. The blood signal suppression within the aortic lumen allows an optimal evaluation of the vessel wall, with a proper definition of the intimal flap on the neck vessels (a arrowheads) and correct identification of the thrombus within the aortic false lumen (b,c arrows), which is hyperintense due to the absence of the normal flow-void effect
Bright-Blood Sequences
This type of sequence is used for the evaluation of the vascular lumen, but with less definition of the vessel wall compared to dark-blood acquisitions. In addition to the sequences whose use is well established in the MRA studies (TOF and PC), the so- called balanced sequences also belong to this group of techniques. This type of sequence uses the residual transverse magnetization of the moving blood protons subjected to very low TR RF pulses, and amplifies it through the use of additional gradients, thus allowing fast acquisition with a high intravascular signal (Fig.2.8). However, the use of these sequences without the contemporary application of cardiac trigger, does not allow adequate differentiation between arterial and venous structures, reducing their current effective applicability in some scanners.
ToF Sequences
The ToF sequences are based on the application of the in-flow effect. Currently, these sequences are used as the first diagnostic choice in the evaluation of intracranial circulation and as a second option for the study of epiaortic vessels, when CE-MRA can’t be performed. The ToF sequences, despite the high spatial resolution, are in fact very sensitive to motion artifacts due to respiration or long acquisition times; they are also very sensitive to artifacts due to protons dephasing with loss of the signal intensity caused by very slow flow velocity as observed in cases of severe stenosis or tortuous vessels, with the consequent risk of overestimation of the degree of stenosis (Fig.2.9). MRA with ToF sequences can be performed with 2D or 3D acquisitions.
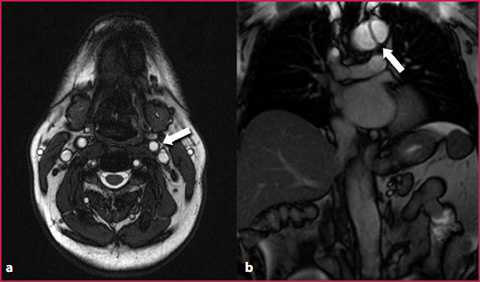
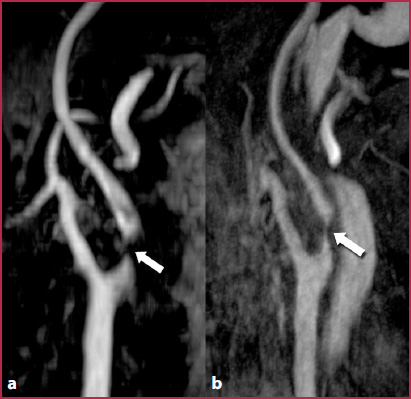
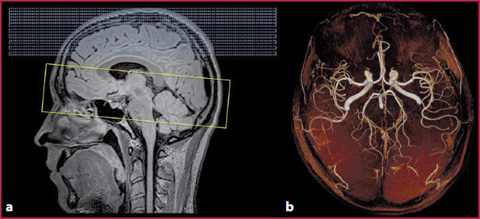
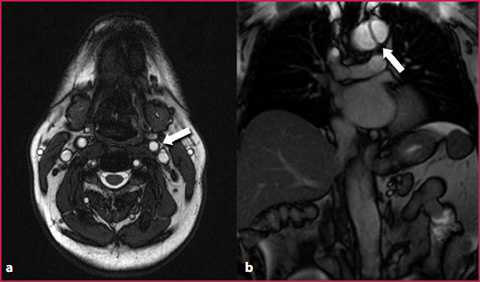
Fig. 2.8
Images obtained with the balanced bright-blood technique in a patient with aortic dissection. a Good evaluation of the vascular lumen, but without adequate differentiation between veins and arteries (arrow). b The intimal flap is very clearly delineated in the thoracic aorta with aortic arch extension (arrow)
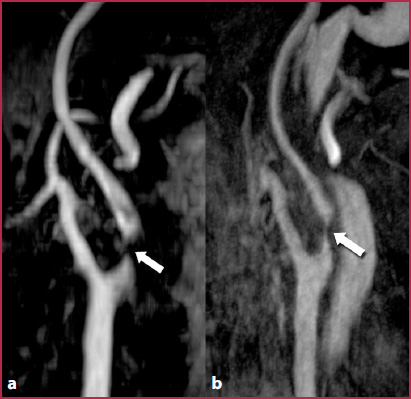
Fig. 2.9
ToF images obtained in a patient with carotid stenosis. a The morphology and the course of the vessel is correctly evaluated, as well as the plaque evident at the origin of the left internal carotid artery. However, the extremely slow and turbulent flow at the site of the stenosis leads to dephasing of the blood protons, with loss of the in-flow effect and absence of the signal within the arterial lumen (arrow), with a consequent overestimation of the degree of stenosis compared to the evaluation in the contrast-enhanced images (b), in which proton dephasing artifacts are not present at the level of the plaque (arrow)
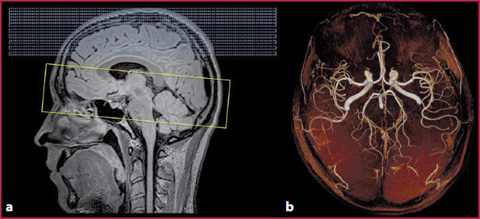
Fig. 2.10
a Perpendicular position of the acquisition volume in relation to the long-axis of the vessel in a ToF sequence (in yellow), used in this case for the study of intracranial arterial circulation. The use of a band of saturation (in blue) applied cranially to the volume in analysis enables saturation of the incoming venous flow. b This results in the acquisition of images in which the signal intensity is determined only by the arterial structures
In the past, it has been demonstrated that the diagnostic accuracy of 2D acquisitions is particularly burdened by the previously described proton dephasing artifacts, which often determine an overestimation of the degree of stenosis in the presence of severe vessel stenosis, especially in the carotid examination. The introduction of the 3D ToF sequences with a thin slice thickness and the multiple overlapping thin slab acquisition (MOTSA) resulted in a noticeable increase in the spatial resolution and diagnostic accuracy of this technique, making it a potential alternative to CE-MRA in selected patients. A technically adequate ToF acquisition needs to follow some mandatory steps:
the acquisition plane must be perpendicular to the vessel and to the flow under examination (Fig.2.10);
the application of selected spectral presaturation pulses for the substances with short T1 that can be hyperintense, similar to the blood through the vessels (fat, methemoglobin and blood clots);
the use of 2D ToF sequences for the study of the venous vessels and of 3D ToF sequences for the arterial vessels;
the application of spatial presaturation pulses (bands of saturation) in order to saturate the incoming flow which has an opposite direction to that that must be displayed and examined (for example, the selective saturation of the venous blood flow to obtain only arterial information or vice versa).
The ToF sequences characteristics are summarized in Table 2.2.
Table 2.2
ToF sequence characteristics
Advantages |
Easy to use |
High spatial resolution |