Fig. 6.1
The skull bone. A complex medium for transcranial ultrasonic propagation
Sound speed within the skull bone ranges between 1500 m/s in the liquid phase of porous regions, and 3000 m/s in dense cortical regions (Fry and Barger 1978). Local density in the skull ranges between 1000 kg/m3 in the liquid phase of porous regions and 2200 kg/m3 in dense cortical regions. The speed of sound spatial heterogeneities strongly varies from one region to another and from one individual to another. A major reason for this high variability is the micro-structured architecture of the skull bone.
The inner and outer tables are defined by a very low porosity with a pore size ranging between 50 and 100 μm. For typical HIFU frequencies (500 kHz to 1.5 MHz), this pore size is very small compared to the ultrasonic wavelength in the bone (~3 mm at 1 MHz) and these cortical layers can be considered as a relatively homogeneous medium. On the contrary, the pore size is much larger in the trabecular layer (diploe) and is not negligible compared to the ultrasonic wavelength. Consequently, the acoustic propagation is influenced by the inner geometry of the skull bone (Aubry et al. 2003). Mode conversion and acoustic diffusion are more significant in the diploe than in inner and outer layers. This leads to a much higher attenuation of the ultrasonic beam in this inner region of the skull. In the classical frequency domain of transcranial HIFU (300 kHz to 1 MHz), the skull attenuation is due to both acoustic diffusion (reversible phenomenon) and absorption (irreversible phenomenon). The part of ultrasonic attenuation resulting from acoustic diffusion is more significant than that resulting from absorption (Fry and Barger 1978; Pinton et al. 2012a).
This complex medium introduces both strong phase aberrations and attenuation on the ultrasonic beam during its transcranial propagation. These aberrations have been extensively explained by Fry and Barger, but have remained a bottleneck for non-invasive transcranial therapy for several decades. To compensate for these distortions, Phillips et al. (1975) introduced for diagnostic applications the idea of adaptive focusing by applying a correcting phase on the transmit signal of each element of the ultrasonic array. This concept has been adapted for therapeutic applications, as presented in the following section.
6.2 Skull Aberration Correction Techniques
6.2.1 Minimally Invasive Correction
In 1996, Thomas and coauthors introduced the concept of time reversal mirror for transcranial HIFU therapy (Tanter et al. 1996; Thomas and Fink 1996). They proposed embedding a miniature hydrophone on the surgeon’s catheter used during the biopsy. Then by spatial reciprocity they recorded the signal transmitted from a point located close to the targeted area (tumor) to the HIFU array. At the end of the minimally invasive biopsy exam and after removing the catheter, the HIFU device would be used if the tumor malignancy was confirmed. In order to perform adaptive focusing of the ultrasonic therapeutic beam, the HIFU system would emit the time-reversed version of the initial experimental signals acquired during the biopsy. In conjunction with the phase aberration correction performed by the time reversal process, an amplitude compensation was also proposed in order to correct for both speed of sound and absorption aberrations caused by the skull. Using this time reversal focusing concept, they demonstrated both a 10 dB improvement of the focusing quality, and the ability to focus at the initial target location, thus cancelling the shifts induced by the skull. Initially limited to ultrasonic adaptive focusing at the sole location of the initial miniaturized hydrophone, this concept was extended in 1998 by Tanter et al. to adaptive focusing in large regions around the initial autofocus point (Thomas and Fink 1996; Tanter et al. 1998). In order to electronically steer the beam and heat the whole target spot by spot without moving the therapeutic array, the authors proposed combining the time reversal process with a numerical back-propagation and steering algorithm. They experimentally demonstrated the feasibility of this approach on ex-vivo human skull bones.
In 1998, Hynynen and Sun numerically modeled a phase conjugation technique (equivalent to the time reversal process for phase aberration correction of monochromatic signals) with a high intensity ultrasound system (Sun and Hynynen 1998). In 2000 and 2002, Clement et al. evaluated this phase conjugation technique from a single point location and extended the sonication regions around the initial focus by using a steering technique equivalent to the one introduced by Tanter et al. Clement et al. validated this approach at high power by performing in–vitro necrosis at the geometrical center of a spherical transducer array using a therapeutic beam steered from an implantable hydrophone located 1 cm away from geometrical focus (Clement and Hynynen 2002b). In 2004, Pernot et al. performed a first preclinical trial on 20 sheep using the time reversal focusing concept (Pernot et al. 2004, 2007). Transcranial adaptive focusing was achieved using a 300-element HIFU array driven by a fully programmable system with 300 independent electronic channels. The histological examination confirmed that thermal necrosis had been induced in the targeted area and that no skin and skull burns occurred.
6.2.2 Non Invasive Correction
Some years later, the possibility to assess the acoustic properties of each patient skull from MRI (Clement and Hynynen 2002a, b) or CT images (Aubry et al. 2003) raised new hopes for fully noninvasive brain therapy. Thus, the minimally invasive procedures described in (Tanter et al. 1998, Clement and Hynynen 2002) were replaced by a totally non-invasive method guided by imaging modalities to predict skull aberrations. Hynynen et al. proposed MRI guidance (Clement and Hynynen 2002a, b) for extracting the skull profile without information on internal heterogeneities. Although this simplified model improves the focusing beam quality, it is not sufficient to completely model the phase aberrations induced by the skull. Heterogeneous speed of sound and density maps can be extracted from high-resolution CT images. For this reason, CT imaging is more suited for modeling the ultrasonic properties of the skull (Pernot et al. 2003; Marquet et al. 2006).
Aubry et al. (2003) suggested and validated a way to extract local acoustic properties of the skull from 3D CT scans and then used these parameters in a numerical model of the wave propagation based on three-dimensional finite differences. By taking into account the internal bone structure (heterogeneities in density, speed of sound and ultrasonic absorption coefficient), this finite difference simulation models all the defocusing effects first highlighted by White et al. (1968). The feasibility of such transcranial ultrasound therapy based on the prior acquisition and guidance of 3D CT-scans was confirmed in-vitro, and the performances of the fully adaptive focusing protocol was studied by Marquet et al. (2006). They demonstrated that this focusing approach based on 3D CT scans and numerical modeling was reaching an excellent focus localization with a spatial variance of the focal spot being of the order of 1 mm (i.e. comparable to the CT scan voxel size). The same system was used to perform preclinical demonstration of such noninvasive CT-based HIFU treatment on 5 monkeys (Tanter et al. 2007; Marquet et al. 2013). Monkeys were sacrificed 2 weeks after the treatment and no skin burn was reported. The histological examination confirmed thermal acidophilic necrosis in the area treated with the CT based time reversal correction, whereas no necrosis was induced in regions without adaptive focusing. The first clinical application of CT-based adaptive focusing of ultrasonic therapeutic beams was performed on 11 patients suffering from chronic therapy-resistant neuropathic pain during TcMRgFUS-induced thalamotomy (Martin et al. 2009).
In conclusion, adaptive focusing techniques for transcranial ultrasonic therapy are based on time-reversal processing (Thomas and Fink 1996; Tanter et al. 1998) or phase conjugation focusing (Sun and Hynynen 1998; Clement and Hynynen 2002a, b), and have been shown to restore a good focusing quality down to 20 dB, which is known to be sufficient for ultrasonic therapy. Two kinds of correction algorithms are used: 3D finite difference simulations of the full propagation through the skull bone structure for the time reversal approach (Aubry et al. 2003), and a layered wavevector-frequency estimation for the phase conjugation technique (Clement and Hynynen Clement and Hynynen 2002a, b). The focusing quality was further improved down to 40 dB by correcting both phase and amplitude thanks to spatio-temporal inverse filtering (Tanter et al. 2000; Aubry et al. 2001; Tanter et al. 2001; Vignon et al. 2006) for future optimal transcranial brain imaging.
Recently, another adaptive focusing approach was proposed that does not require the use of numerical modeling (Herbert et al. 2009; Larrat et al. 2010). Correction of the therapeutic beam is performed by maximizing the local displacement induced at the targeted location by the radiation force of transmitted ultrasonic signals, as imaged by MR-Acoustic Radiation Force Imaging (Hertzberg et al. 2010; Larrat et al. 2010; Kaye et al. 2011; Marsac et al. 2012). To date, this approach was validated only in human cadaver heads (Marsac et al. 2012) and further clinical translation requires further developments. If successful, this approach could be of great interest, as it does not rely on any numerical modeling of transcranial propagation, but rather on the direct observation and optimization of acoustic beam intensity using MRI.
6.3 Thermal Therapy
The technological developments presented in previous sections have led to the development of two brain therapy devices. The devices have been first tested on cadaver (Marsac et al. 2012; Chauvet et al. 2013; Monteith et al. 2013b), as illustrated in Fig. 6.2, and have been recently translated into clinics for non-invasive TcMRgFUS thalamotomy (see next section). Current research focuses on expanding the treatment envelope to other areas of the brain, as presented later in this chapter.
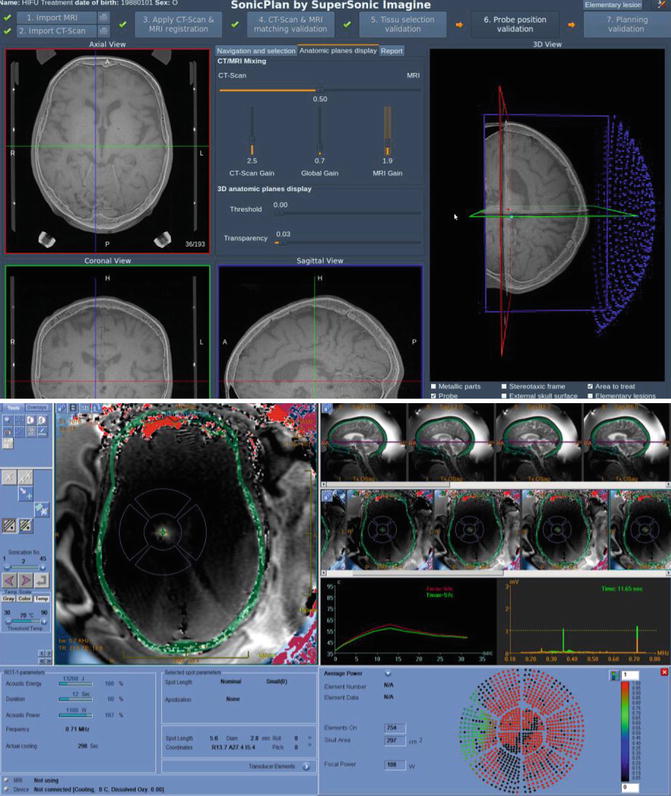
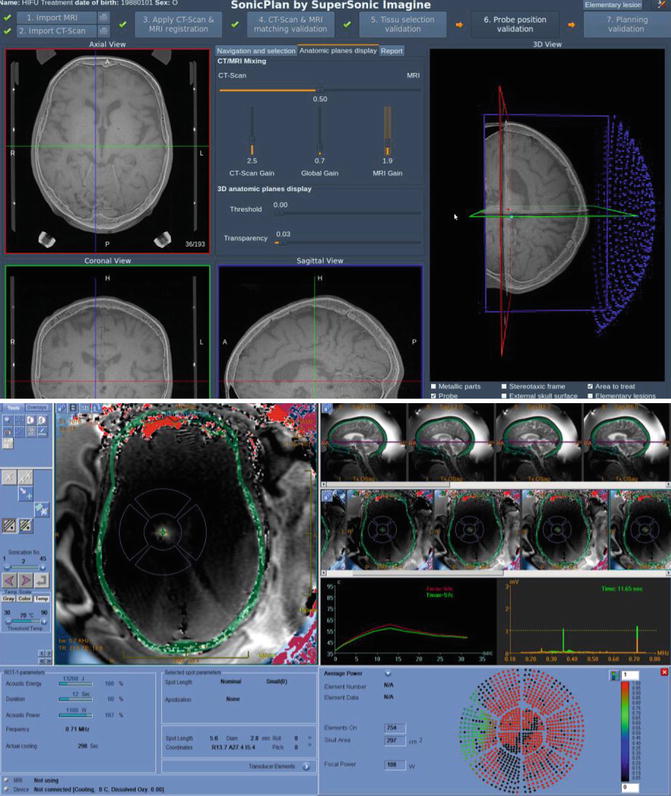
Fig. 6.2
SuperSonic Imagine (Top) and Insightec (Bottom) planning and monitoring software during planning (Top) and monitoring (Bottom) phase of human cadaver experiments
6.3.1 Thalamotomy
The thalamus is a neurological relay that plays a major role in many movement disorders such as Essential Tremor, Parkinsonian tremor or Dyskinesia, but also in neuropathic pain. It is deeply seated in a central area of the brain and is thus an ideal candidate for TcMRgFUS from an ultrasonic point of view given it allows a high antenna gain (ratio between the surface of the beam intercepting the skull and the transverse surface of the beam in the focal plane).
Surgical intervention was proposed in the 70s to address tremor with radiofrequency lesioning or electrical stimulation of the ventralis intermediate nucleus of the thalamus (Tasker 1998). Radiofrequency ablation in the medial thalamus also showed long term reduction of neurogenic pain (Jeanmonod et al. 2001). Nevertheless, in both cases patients are reluctant to undergo such invasive surgeries. Targeting the thalamus with focused ultrasound is thus of great potential. This was actually demonstrated in-vivo in pigs (Elias et al. 2013b). This study showed that long sonication (typically 10–30 s) of high power focused ultrasound (typically 1 kW/cm2 at focus) can locally increase the temperature and induce thermal necrosis that evolves similarly to radiofrequency lesions, as assessed by MRI, histology and theoretical modeling (Elias et al. 2013a, b).
Example – Neuropathic pain
The first TcMRgFUS-induced thalamotomy was performed in Zurich on 11 patients suffering from chronic therapy-resistant neuropathic pain. Short term outcomes of the first 9 patients were reported in a preliminary paper (Martin et al. 2009) and a complete report was published with two additional patients (Jeanmonod et al. 2012). Peak temperatures of 51 and 53 °C were achieved in the first two patients in the posterior part of the central lateral thalamic nucleus, as measured by MR-based temperature mapping. The acoustic power was increased for patients 3–9, and the peak temperature ranged between 51 and 60 °C. Power was further increased and a peak temperature of 64 °C was achieved in the last two patients. All patients experienced somatosensory and vestibular manifestations during sonication, but only six patients experienced immediate and persisting somatosensory improvements. Sensory improvements were not correlated to the peak temperature at focus (Table 6.1). The precision of the targeting was further investigated in (Moser et al. 2012). The authors computed the global accuracy as the difference between the coordinates of the center of the lesion as seen on post-operative MR images and the coordinates of the target as defined on the pre-treatment MR coordinates of the target. The mean global error was 0.54 ± 0.34 mm in the antero-posterior direction, 0.72 ± 0.42 mm in the medio-lateral direction and 0.72 ± 0.39 mm in the dorso-ventral direction. In 45 % of the patients (patients #1, #2, #3, #7 and #11) the error along one axis exceeded 1 mm. Thus, lack of sensory improvement does not correlate with largest targeting errors.
Table 6.1
Summary of thalamotomy treatments using focused ultrasound for neuropathic pain
Case number | Max temperature at target (°C) | Sensory improvements | Side effects |
---|---|---|---|
1 | 53 | + | |
2 | 51 | + | |
3 | 57 | + | |
4 | 59 | – | |
5 | 55 | + | |
6 | 58 | + | |
7 | 57 | – | |
8 | 55 | – | |
9 | 60 | – | |
10 | 64 | + | |
11 | 64 | – | a |
Overall | 57.5 |
Right after the last sonication of the treatment, patient #11 suffered an acute appearance of right-sided motor hemineglect with dysmetria of the arm and leg and dysarthria (Jeanmonod et al. 2012). Post-treatment MR images showed a 10 mm bleed centered in the targeted thalamic central lateral nucleus, as well as ischemic changes in the posterior part of the thalamic motor ventral lateral nucleus. An 80 % reduction in the motor symptoms were reported over the next 24 h and dysmetric manifestations almost disappeared, except during activation of the finer functions of speaking and writing.
Bleeding can be induced by the violent collapse of ultrasound-induced bubbles created during sonication by a process called acoustic cavitation (Leighton 1994). The brain is very sensitive to such collapse and hemorrhagic effects have been reported in dog brains by Fry et al. (1995) at the cortical gray and subcortical white matter interface when multiple adjacent focal sites where exposed to ultrasound. Acoustic emission induced by the collapse of cavitation bubbles can be detected by passive cavitation detectors and passive mapping can be sensitive up to single cavitation events (Gateau et al. 2011a). Passive cavitation detection can thus be used to trigger a shutdown of the system at the early onset of cavitation (Gyongy and Coussios 2009). Following the adverse event reported in (Jeanmonod et al. 2012), passive cavitation detection was implemented on the Exablate 4000 system for upcoming clinical trials.
Example – Essential Tremor
Between 2011 and 2012, 15 drug-resistant patients participated in a phase I study on essential tremor with the Exablate 4000 system at the University of Virginia (Elias et al. 2013a, b). The treatment consisted of a controlateral thalomotomy of the ventralis intermediate nucleus (VIM). A series of low-power sonications were delivered to the intended target to validate the location of the target. Once verified, thermal rise was achieved until the measured thermal dose was higher than 240 cumulative equivalent minutes (CEM). The maximum temperatures reached ranged between 55 and 63 °C. The results showed an average decrease of the tremor of 67 % 1 year after treatment, resulting in a reduction of disability of 83 %. However, a mild facial paralysis and numbness of the fingers were observed in some cases (transiently for 9 patients, and persistent after 1 year for 4 patients). At the end of 2012, four patients with essential tremor of the hand were also treated in Toronto (Lipsman et al. 2013). The targets were in the VIM opposite to the most affected hand. The focus was verified by moderate heating and adapted so as not to affect the adjacent sensory areas. The lesions were gradually extended by increasing the power or duration of emissions (between 12 and 29 times in increments between 0 and 2 °C and between 10 and 25 s) until the disappearance of tremor or occurrence of adverse effects. Temperatures between 56 and 63 °C were reached. The tremors decreased significantly during treatment and after 3 months. However, paresthesia at the tip of the thumb occurred in one patient during treatment, still persisting after 3 months. Another patient had a deep vein thrombosis, which, according to the authors, was potentially linked to the duration of treatment. More recently, 11 patients were treated at Yonsei University College of Medicine, Seoul, Korea (Chang et al. 2014), showing an overall significant decrease in tremor symptoms (Table 6.2), but treatment was not possible for three patients: The maximum temperature at target was less than 42 °C, even with up to 24 000 acoustic joules emitted by the transducer (1200 W during 20 s).
Table 6.2
Summary of essential tremor treatments with focused ultrasound
Reference | Case number | Max temperature at target (°C) | Sensory improvements | |
---|---|---|---|---|
Baseline CRST (total) | 3 months CRST (total) | |||
Lipsman et al. (2013) | 1 | 56 | 63 | 28 |
2 | 63 | 82 | 42 | |
3 | 59 | 47 | 24 | |
4 | 59 | 91 | 47 | |
Overall Mean | 59.25 | 70.75 | 35.25 | |
1 | N/A (55–63) | N/A average: 55 | N/A average: 25 | |
2 | N/A (55–63) | N/A average: 55 | N/A average: 25 | |
3 | N/A (55–63) | N/A average: 55 | N/A average: 25 | |
4 | N/A (55–63) | N/A average: 55 | N/A average: 25 | |
5 | N/A (55–63) | N/A average: 55 | N/A average: 25 | |
6 | N/A (55–63) | N/A average: 55 | N/A average: 25 | |
7 | N/A (55–63) | N/A average: 55 | N/A average: 25 | |
8 | N/A (55–63) | N/A average: 55 | N/A average: 25 | |
9 | N/A (55–63) | N/A average: 55 | N/A average: 25 | |
10 | N/A (55–63) | N/A average: 55 | N/A average: 25 | |
11 | N/A (55–63) | N/A average: 55 | N/A average: 25 | |
Baseline CRST (A + B + C) | 6 months CRST (A + B + C) | |||
Chang et al. (2014) | 1 | N/A (48–61) | 34 | 8 |
2 | N/A (48–61) | 22 | 1 | |
3 | N/A (48–61) | 36
![]() Stay updated, free articles. Join our Telegram channel![]() Full access? Get Clinical Tree![]() ![]() ![]() |