MR of Fetal Brain and Spine
Daniela Prayer
Gregor Kasprian
Christian Mitter
Gerlinde M. Gruber
Peter C. Brugger
The use of magnetic resonance imaging (MRI) to improve the in utero diagnosis of fetal cerebral and spinal pathologies has become increasingly important since the availability of ultrafast sequences and parallel imaging that have eliminated the need for maternal sedation or fetal curarization (1,2,3). Indications for MR examination of the fetal central nervous system (CNS) are usually defined after a prior ultrasound (US) study (4). It remains true that US, due to wider availability, lower costs, and easier applicability, remains the primary imaging modality during pregnancy, but MR may provide information that cannot be acquired by sonographic methods (Table 26.1) (5,6). Morphologic imaging remains the mainstay of MR diagnosis, but other modalities, such as tractography, spectroscopy, and functional imaging, are currently being evaluated for their possible contributions to improve diagnostic and prognostic accuracy in CNS pathologies (7,8,9). Because of the signal differences between cerebrospinal fluid (CSF) (bright) and brain tissue (dark), T2-weighted contrast has been primarily used to describe the surface of the fetal brain (10). Improvement of sequences and sequence parameters now enable a more detailed assessment of the brain tissue and its diseases, not only by T2-weighted contrast, but also using T1-weighted, diffusion-weighted, and FLAIR sequences. Diffusion tensor imaging (DTI) may be used for further tissue characterization and for tractography (11,12). Echo-planar imaging (EPI) sequences add information about hemorrhage and/or calcifications and blood-oxygen-level-dependent (BOLD) imaging can be used for functional imaging studies. Single-voxel spectroscopy may be performed to give insight into brain metabolism (13). MRI of the spinal cord relies mainly on T2-weighted contrast that allows delineation of the cord from the surrounding CSF. In addition, EPI sequences show bone pathologies, and T1-weighted sequences may visualize spinal fat after gestational week (GW) 27/28 (14).
As with MRI, ultrasound methods have also experienced advancements during the last few decades. Resolution has improved, and 3D and multiplanar imaging have become available, which may demonstrate subtle anatomical details, especially in the hands of dedicated neurosonographers (15,16). However, MRI visualizes the whole brain, even in late pregnancy, and orthogonal sections can be acquired more easily, as shadowing by the bony skull, which requires complicated angling of ultrasound probes, does not impair MR image quality.
For fetal CNS, MRI is currently used successfully in the assessment of brain malformations, especially those associated with abnormal cortical development (17), in commissural and infratentorial malformations. Acquired pathologies, such as infarctions and hemorrhages, can be detected sensitively. In addition, spinal malformations can be described more accurately than with ultrasound alone, which has become important because of the increasing application of intrauterine surgical repair of open spinal defects (18). In any case, it is necessary to examine not only the fetal CNS, but also the extra-CNS parts of the fetus, and to look at the extrafetal organs, especially at
the placenta. Otherwise, complex syndromes and/or acquired conditions, such as infections, may be misdiagnosed.
the placenta. Otherwise, complex syndromes and/or acquired conditions, such as infections, may be misdiagnosed.
TABLE 26.1 Relative Values of Fetal Ultrasound Compared to Fetal MRI, Subjective Estimate | |||||||||||||||||||||||||||||||||||||||||||||||||||||||||||||||||||||
---|---|---|---|---|---|---|---|---|---|---|---|---|---|---|---|---|---|---|---|---|---|---|---|---|---|---|---|---|---|---|---|---|---|---|---|---|---|---|---|---|---|---|---|---|---|---|---|---|---|---|---|---|---|---|---|---|---|---|---|---|---|---|---|---|---|---|---|---|---|
|
In recent years, fetal postmortem MRI studies have gained importance, especially with the possibility to use field strengths higher than 1.5 T, because the performance of pathologic work-ups by fetopathologic specialists has decreased worldwide. In case of a failure to make a diagnosis intra vitam, postmortem MRI offers the investigation of the organs in situ, which may play a role in case of intracranial cystic pathologies that may change in appearance after dissection. Consequently, the postmortem MRI may represent the only opportunity to achieve a definitive diagnosis, which is especially important in pathologies with a possible genetic background (19,20).
Safety Issues
There are no known biologic risks from MRI. The ACR Guidance Document on MR Safe Practices 2013 states that “pregnant patients can be accepted to undergo MR scans at any stage of pregnancy” if the medical situation warrants this examination (21). The ACOG Guidelines for Diagnostic Imaging, reaffirmed in 2009, say that MRI is not associated with known adverse fetal effects and that “this technique could prove especially useful for diagnosis and CNS anomalies.” Neither statement makes any reference to field strength. However, the Report of the Canadian Task Force on Preventive Health Care concludes that “Fetal Magnetic Resonance Imaging is safe at 3.0 Tesla or less during second and third trimesters (22). Gadolinium crosses the placenta and appears within the fetal bladder only moments after intravenous administration. From the fetal bladder the contrast is excreted into the amniotic fluid, where it is then swallowed and potentially reabsorbed from the gastrointestinal tract. In clinical practice, the use of Gadolinium as a contrast medium is encouraged when the benefits outweigh the potential risks” (21,22).
3 T Fetal MRI
The use of higher field strengths than 1.5 T has been shown to provide the ability to scan faster and/or provide more information about small anatomical details (23). However, imaging of the pregnant abdomen is still a challenge, as artifacts, especially resulting from amniotic fluid, have to be overcome (24). Experience with 3 T fetal MRI is still limited, but improved signal intensity and resolution, compared with 1.5 T has been shown (25). In addition, certain applications, such as spectroscopy (26) or functional MRI (fMRI) (27), seem to benefit from higher field strength.
Sequences for Fetal MRI
Imaging of the fetal brain and spine heavily relies on T2-weighted turbo spin-echo (TSE) sequences. A set of continuous images in three orthogonal planes of the fetal head depicts normal and pathologic anatomy. Dealing with small structures, an in-plane resolution of approximately 0.8 mm/pixel, and a slice thickness of 3 mm is recommended whenever possible, especially in fetuses younger than 24 GW. Thicker slices (4 mm, 0.4-mm gap) may be used in later gestation, which also minimize the duration of the acquisition. To obtain high-quality images, it is necessary that the region of interest (fetal head or spine) is in the center of the coil. Maternal breath-hold may be indicated when the fetus is in breech presentation, because otherwise images will be degraded by maternal breathing movements.
Steady-state free-precession sequences (SSFP) depict the fetal brain equally well, although the contrast within the cerebral parenchyma may depend on the sequence parameters used.
T1-weighted contrast is provided by fast field-echo sequences. Again, thinner slices (4 mm, no gap) should be used in second-trimester fetuses. Usually, 16 images (either 4 mm thick, or 5 mm with 0.5-mm gap) are sufficient to cover the brain in the axial plane, and can thus be acquired within a single breath-hold.
In addition, many other sequences are available for prenatal MRI (28). EPI demonstrates the osseous anatomy of the skull and spine, and supplies information on the presence of blood-breakdown products. Diffusion-weighted imaging (DWI) with a b-value of 700 visualizes internal brain architecture as well as ischemic lesions (29). The feasibility of DTI has been demonstrated in utero (11) are instrumental in assessing disorders of connectivity.
Although single-voxel spectroscopy with 128 acquisitions lasts for nearly 5 minutes, it yields readable spectra in two-thirds of cases, even when used without prior sedation of the mother and fetus (30).
Normal Fetal Brain Development
Normal fetal brain development is characterized by an increase of tissue starting from about 20 GW (31), progressive cortical folding, and a change in the laminated appearance of brain tissue into the structured gray/white matter regional organization encountered in mature brains (Fig. 26.1) (32,33). In addition, asymmetric growth of the cerebral hemispheres can be observed as a morphologic landmark of normal brain development (34). A thorough knowledge of the appearance of the fetal brain in a specific GW is essential for recognizing pathologies already present in the second trimester. Fetal brain development can also be demonstrated using atlases that are calculated from fetal MR by segmentation of anatomical structures, which allow discovery of deviations from normal brain development by comparison with the calculated model with combined data from a cohort of normal individuals (35,36). White matter development may be visualized using diffusion tensor–based tractography (11). In addition, normal fetal brain development is characterized by nonmorphologic changes, such as the emergence of functional networks that may be demonstrated using resting-state fMRI (8,27). Metabolic changes of the developing fetal brain may be studied using proton spectroscopy (37).
Fetal movements have been interpreted as the output of the maturing cerebral structures (38). While so-called general movements can be observed both qualitatively and quantitatively using ultrasound (39), qualitative assessment of special intrinsic movement patterns that evolve over time may be visualized only by dynamic MR studies (40).
Fetal CNS Anomalies
Ultrasound, with its high-resolution images and relatively low costs, is still the primary screening method for fetal anomalies (41). However, MRI has been shown to be advantageous with respect to clinical counseling of cases with CNS malformations (Figs. 26.2 and 26.3), even in a tertiary referral center (4). A systematic review of the literature showed the equality or superiority of MRI compared to US in 87.5% of fetal CNS pathologies, and an inferiority to US in only 2%, with a pooled MR sensitivity of 95% and a pooled specificity of 70% (41). CNS pathologies that may be described in a more detailed fashion by additional MRI comprise disorders of cortical development, commissural malformations, ventricular abnormalities, infratentorial malformations, complex malformations that may include extra-CNS pathology, and acquired changes, especially with hemorrhagic components.
Disorders of Cortical Development
Histologically, cortical development starts in the 8th to 9th postconceptional week (32) (corresponding to the 9th to 10th postmenstrual weeks). Consequently, disorders of cortical
formation are already present at a time when fetal MRI can be performed (usually after 18 GW). Disorders that involve large regions of the cortex, such as lissencephalies (Fig. 26.4) and hemispheric manifestations of polymicrogyria, may be detected by a disordered lamination of the fetal brain, especially by a failure of delineation or an atypical width of the subplate. In addition, the preterm appearance of gyri or atypical sulcation may point toward the presence of abnormal gyration (Fig. 26.5) (42). Furthermore, early hypoxic–ischemic lesions may become clefts, lined by polymicrogyric cortex (Fig. 26.6). In these instances, sensitivity for the detection of polymicrogyria was reportedly 85%, and specificity 100%. For schizencephaly, sensitivity and specificity were each 100%, and 73% and 92%, respectively, for heterotopia (17). Heterotopia may be unidentifiable before the third trimester.
formation are already present at a time when fetal MRI can be performed (usually after 18 GW). Disorders that involve large regions of the cortex, such as lissencephalies (Fig. 26.4) and hemispheric manifestations of polymicrogyria, may be detected by a disordered lamination of the fetal brain, especially by a failure of delineation or an atypical width of the subplate. In addition, the preterm appearance of gyri or atypical sulcation may point toward the presence of abnormal gyration (Fig. 26.5) (42). Furthermore, early hypoxic–ischemic lesions may become clefts, lined by polymicrogyric cortex (Fig. 26.6). In these instances, sensitivity for the detection of polymicrogyria was reportedly 85%, and specificity 100%. For schizencephaly, sensitivity and specificity were each 100%, and 73% and 92%, respectively, for heterotopia (17). Heterotopia may be unidentifiable before the third trimester.
![]() FIGURE 26.2 Alobar holoprosencephaly with monoventricle in 33-week GA fetus on midsagittal (A) and axial (B) T2-weighted images. |
Ventricular Abnormalities
Ventriculomegaly, diagnosed by ultrasound, refers to an atrial width more than 10 mm, with up to 12 mm considered “mild,” up to 15 mm considered “moderate,” and greater than 16 mm considered “severe” ventriculomegaly (43). Whether MRI can contribute important supplementary information in such cases is currently the subject of some controversy (44,45,46). However, as up to 85% of cases with ventriculomegaly have an additional brain pathology (47), MRI might be helpful in further classification of associated changes. “Red flags” in ventriculomegaly (indicating that it is not an isolated finding) are:
irregular borders (51);
intraventricular material (52);
additional brain pathology, such as commissural agenesis/dysgenesis, disorders of cortical formation, malformation of the posterior fossa and/or midbrain, intracranial hemorrhage, thinning of the cerebral mantle;
failure of proof of the presence of a ventricle, as, for instance, obliteration of the fourth ventricle in Chiari II malformation.
Commissural Abnormalities
With a prevalence of 0.02% to 0.5% (53), “callosal agenesis” is frequently encountered in the general population. Today, the complete or partial absence of the corpus callosum can be reliably detected as early as 20 GW or earlier by standard prenatal screening ultrasound (54). Due to the wide variations in neurodevelopmental outcome, ranging from mild neuropsychological
deficits (55) to severe mental retardation (56), personalized prognostic counseling is extremely challenging in these cases. This prognostic uncertainty can be partly attributed to the lack of a standardized postnatal developmental assessment in the present literature (57) and—most importantly—to inconsistencies in the morphologic description of callosal abnormalities.
deficits (55) to severe mental retardation (56), personalized prognostic counseling is extremely challenging in these cases. This prognostic uncertainty can be partly attributed to the lack of a standardized postnatal developmental assessment in the present literature (57) and—most importantly—to inconsistencies in the morphologic description of callosal abnormalities.
![]() FIGURE 26.4 A: Frontal SSFP image of a fetus at 26 GW with lissencephaly. B: Frontal T2-weighted image of the same fetus at 31 GW. |
Showing a mean length between 20 mm at 20 GW and 43 mm at 38 GW (measured by MRI) (58), the fetal corpus callosum is a relatively small structure, which is difficult to resolve with any prenatal imaging technique. However, in order to reach a specific diagnosis, a detailed description of the prenatal brain morphology and the precise characterization of the callosal and commissural abnormality are major prerequisites. As “prototypic” disorders of axon guidance (59), commissural abnormalities frequently may share common genetic origins (60) or can be acquired in cases of hemorrhage, infection, ischemia, and encephalopathies, which can be induced by toxins.
The benefits of fetal MRI in the extended work-up of suspected commissural abnormalities are well acknowledged (61,62,63,64). Currently, fetal MRI provides the (1) detection of a callosal abnormality in cases with the referral diagnosis of “ventriculomegaly” (57), (2) confirmation or rejection of the ultrasound referral diagnosis of “callosal agenesis,” (3) detailed morphologic description of the extent (involved commissure, partial/complete [63]) of the commissural abnormality, (4) identification of additional cortical malformations or acquired pathologies (61), and (5) detection of associated brainstem and cerebellar abnormalities, particularly in later gestational stages, where ultrasound is less successful in visualizing the posterior fossa (65).
Callosal agenesis is the final diagnosis in 13% of fetuses demonstrating “ventriculomegaly” (57). To be more specific, in callosal agenesis, the ventricular widening predominantly affects the occipital horns bilaterally and spares the frontal horns of the lateral ventricles. This was originally observed by C. Benda (66) and further described as by Yakovlev and Wadsworth (67) as “colpocephaly.” In addition, this abnormal ventricular morphology is associated with an abnormal hippocampal positioning, resulting in “nonrotated” hippocampi (Fig. 26.7). Using the commonly observed abnormally oriented gyri and sulci of the medial hemispheres (“radiating gyri”) as an indirect sign for callosal agenesis can be misleading, as they may frequently appear normal (Fig. 26.8) or be present only at late gestational stages.
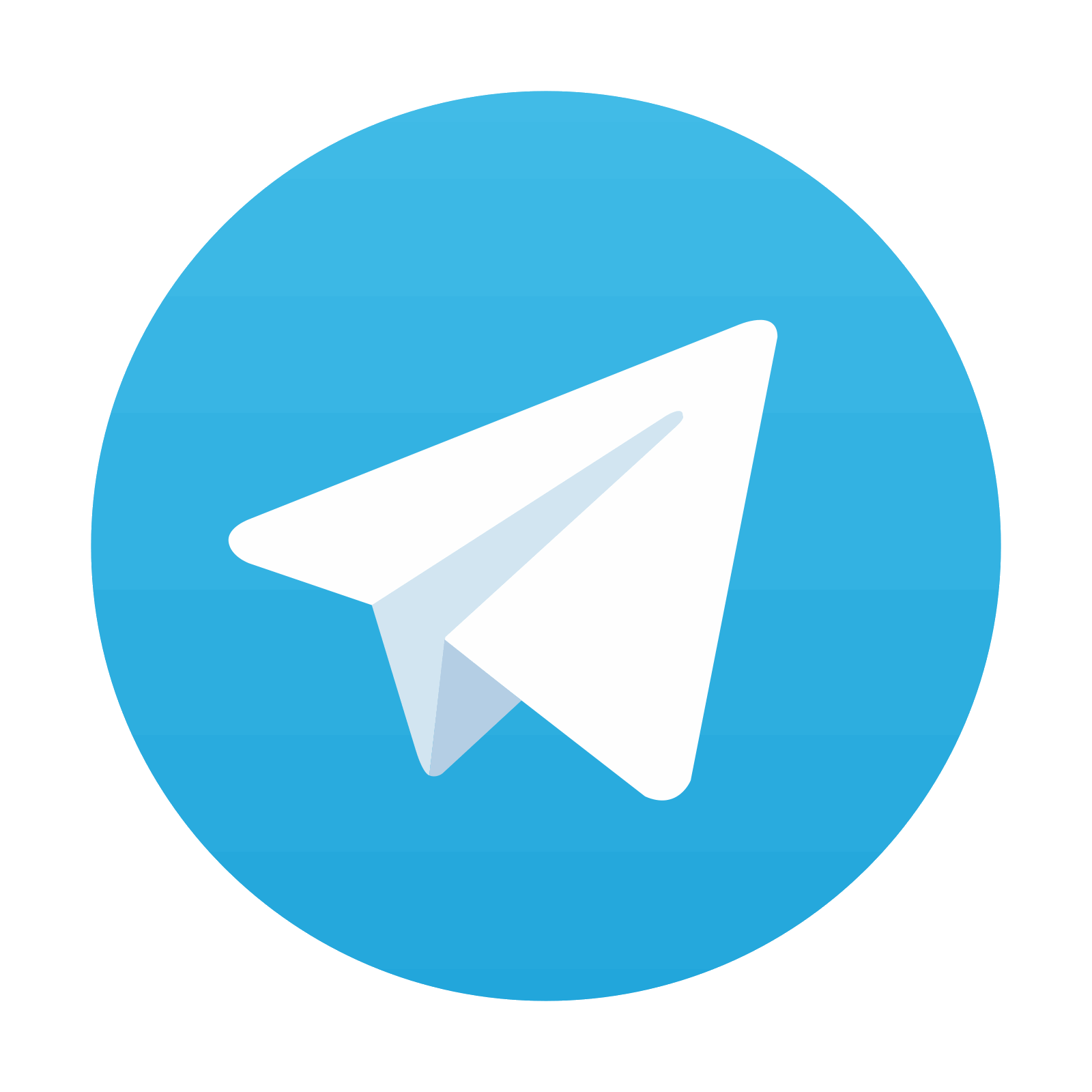
Stay updated, free articles. Join our Telegram channel
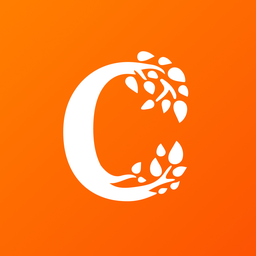
Full access? Get Clinical Tree
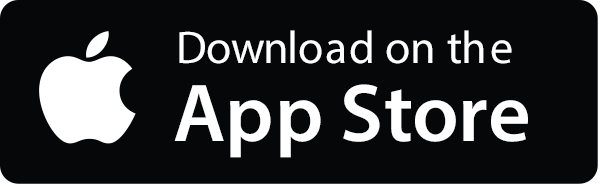
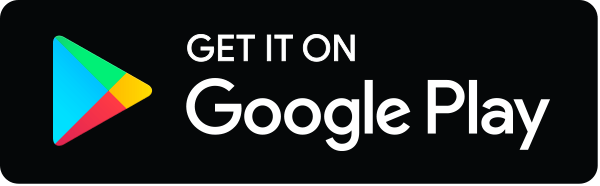