Fig. 28.1
Pre- and post-MRgFUS treatment of uterine fibroid. (a) Sagittal T2W image shows a single dominant uterine fibroid, measuring 5.5 cm. (b) Sagittal T1W image after intravenous administration of gadolinium shows homogeneous nonenhancing necrotic tissue in the area of treatment. (c) Sagittal T2W image 24 months after treatment. (d) Sagittal T2W image at 36 months after treatment. MRgFUS shows continued shrinkage, with the fibroid now measuring 3.8 cm
Breast
The first MRgFUS system built by GE Medical Systems (Milwaukee, WI) was tested by treating benign fibroadenomas in the breast at the Brigham and Women’s Hospital [23] (see Fig. 28.2). Later, the first ExAblate system was introduced by InSightec, and it was tested for the treatment of breast cancer [24]. Surgical removal of breast cancer (lumpectomy) is routinely performed without direct visualization of the tumor and without intraoperative imaging of the definition of tumor boundaries by multiparametric MR imaging. By contrast, in MRgFUS, MR image guidance is expected to be a major advantage as an understanding of both the tumor and its boundaries can be known during surgery so that total ablation of the tumor can be accomplished. In addition this noninvasive alternative to surgical lumpectomy requires no general anesthesia, leaves no surgical scar, and does not require follow-up breast reconstruction.
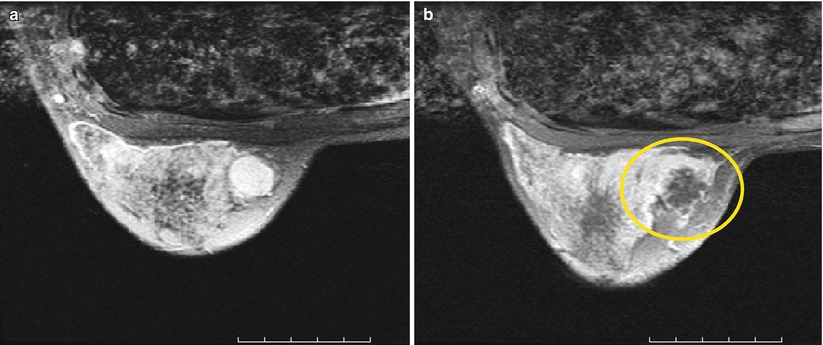
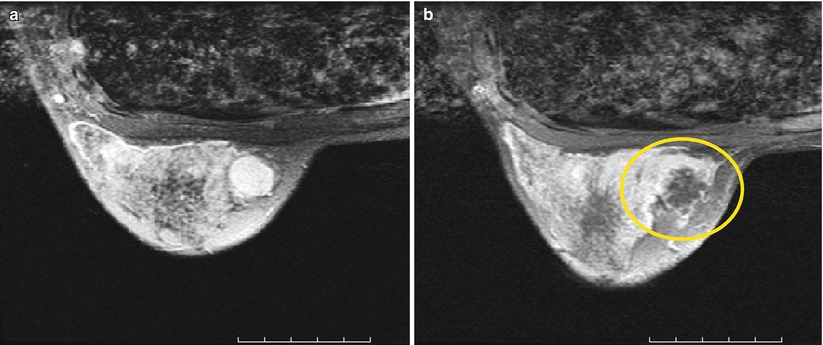
Fig. 28.2
MRgFUS treatment of breast fibroadenoma (a) before (b) immediately after treatment. The coagulation necrosis shows no contrast enhancement
To date, over 200 breast cancer patients have been treated in various clinical trials using MRgFUS. In one series, patients were treated with MRgFUS and then underwent a lumpectomy. Assessment of the resected specimen showed an average necrosis of 97 % with 50 % of the patients achieving 100 % necrosis. These patients then continued their treatment regimen according to the standard of care [25].
Prostate
Due to the widespread use of PSA screening tests, prostate cancer is diagnosed at an earlier age. This early diagnosis, along with the slow progression of prostate cancer, has increased interest in treatment options that can minimize complications. Current treatment options for prostate cancer include prostatectomy (surgical removal of the prostate gland), radiation therapy, brachytherapy (implantation of radioactive seeds), and cryotherapy. These treatment options are accompanied by relatively high levels of adverse events that can significantly impact the quality of life of prostate cancer patients with the most common being incontinence and impotence. FUS ablation for prostate cancer guided by ultrasound imaging has also been used to treat prostate cancer, with promising results. However, reports of side effects associated with the treatment [26] suggest a need for improved treatment planning, monitoring, and guidance. MRI, particularly at 3 T, along with advanced FUS devices that use large-scale phased array devices may offer improvements. The potential advantages of MR guidance over ultrasound guidance are improved imaging of the prostate and its surrounding critical structures (rectal wall, urethral sphincter, neurovascular bundle). Real-time measurement of thermal energy deposition allows for better control of the treatment that can reduce complications. Safe MRgFUS treatment is expected to enable patients to return to normal life within a day or two while maintaining their quality of life. The recently developed ExAblate prostate module is an endorectal device though others have developed intraurethral probes [27]. InSightec initiated early clinical feasibility trials (outside the USA) that started in 2010 that have shown promising results.
Liver
Conventional surgical treatments of liver cancer are invasive and associated with a high incidence of new metastasis and poor success, even after multiple resections or ablations. These limitations motivated research into new, less invasive solutions for liver cancer treatment. Previously, FUS was guided with ultrasound that has limited target definition and monitoring capability of the ablation process. Combining MRI with multiple-element phased array transducers to create MRgFUS for the liver provides more accurate targeting and real-time temperature monitoring. This treatment is hindered by the ribcage that limits the acoustic windows to the liver and the respiratory motion of the liver. New advances in MRI and transducer design will likely resolve these limitations and make MRgFUS a powerful tool in local liver cancer therapy [28].
To date, only a few clinical cases have been performed using MRgFUS by using either respiratory gating or by discontinuing the use of respirator for a short period [28, 29]. Ongoing research is aimed at achieving better temperature monitoring and control of the FUS beam on freely breathing patients who need no general anesthesia [30, 31].
Bone
One of the most successful applications of MRgFUS is the palliative therapy of patients with painful bone metastasis. Over 200 patients with painful bone metastases have been treated. Phase I and II clinical studies have shown that pain scores were reduced from an average of 5.9–3.8 within 3 days after treatment and further reduced to 1.8 at 30 days follow-up. Pain relief was maintained for at least 3 months. Moreover, 72 % of the patients experienced significant pain relief and 50 % experienced complete pain relief [32–34]. An ongoing phase III clinical study for FDA approval is currently being conducted. Regardless of the primary cancer they have, patients with osteolytic as well as osteoblastic lesions can be treated. During a bone treatment, the system is configured so that the targeted bone is in the near field of the FUS beam rather than at the focus as in a soft-tissue treatment. The FUS beam is positioned perpendicular to the bone cortical surface for maximum absorption. During each sonication, the heat absorbed by the bone is capable of ablating the pain fibers in the periosteum resulting in pain relief.
Brain
Since the beginning of the field [35], FUS has been investigated for the treatment of brain disorders. FUS has been investigated for more than 60 years for a variety of therapeutic uses, ranging from producing transient effects, such as temporarily changing physical and functional tissue properties, to tissue ablation [36]. Pioneering work by William and Francis Fry [37] and others showed the huge potential of this technology in animal trials, and promising clinical treatments of neurological disorders were performed [38]. Despite the early promise, the use of FUS, particularly in the brain, has not reached widespread use. The challenge has been due to two main obstacles: a lack of control of the procedure and the difficulty in applying ultrasound through the skull. In recent decades, improvements in medical imaging have led to a resurgence of interest in FUS. The incorporation of ultrasound imaging, which allows some ability to target the beam, and more significantly MRI, which allows for exquisite targeting and feedback control of the procedure with quantitative temperature imaging, has led to several FUS systems designed for a wide range of targets outside of the brain. MRI-based guidance has also been demonstrated for laser thermal ablation in the brain [39, 40]. Despite these developments, the skull has been an obstacle for the use of FUS in the brain. If the ultrasound exposures (“sonications”) were performed through a craniotomy, a noninvasive procedure became invasive and therefore substantially less desirable.
The enormous benefits of applying this noninvasive method in the brain for treating tumors, epilepsy, and movement disorders have been understood for many years, but the need for a craniotomy and the absence of imaging technologies delayed its development. For decades, therapeutic ultrasound through an intact skull was considered impossible due to disruption of the focused acoustic beam and skull heating. Transcranial application of FUS has been prevented because of the interaction of the skull on the ultrasound propagation. Acoustic attenuation in bone, which is ~30–60 times higher than in soft tissue, causes rapid heating in the skull and limits the exposure levels that can be safely applied. A solution to this problem is a hemispherical transducer operating at a lower frequency that generates the ultrasound beam and with which the scalp can be actively cooled. The lower ultrasound frequency reduces absorption in the skull, and the hemispherical design distributes the resulting bone heating over a large enough area to prevent overheating. Its large geometric aperture also increases the gain of the transducer and permits sufficient focal intensity to allow for ablation even at lower frequencies. Simulations and experiments suggest that an optimal ultrasound frequency to balance the skull and focal heating is approximately 700 kHz lower than the 1–4 MHz commonly used in other targets [41]. While lower frequency ultrasound and a hemisphere design can mitigate bone heating, the skull also has a huge effect on the beam propagation. Variations in skull shape and thickness make it impossible to achieve such focusing reliably without some sort of correction using an array of a large number of transducers to tailor the beam propagation [42–44]. With individual driving hardware for each element of this array, one can apply phase offsets to correct for delays in the wave propagation at each location in the skull. The patient-specific corrections required for each element of this phased array can be determined using acoustic simulations combined with the geometric and density information obtained from CT scans of the skull acquired before treatment [44, 45]. Phase offsets can also be used to steer the focal position electronically, and modification of the amplitude of each element can normalize the ultrasound intensity across the brain surface.
Based on extensive preclinical acoustic and MRI [46–48] studies, a clinical prototype of a phased array transcranial MRgFUS device for thermal ablation was developed [49, 50]. InSightec translated this technology to a clinical system (ExAblate 4000) seen in Fig. 28.3. This system utilizes a hemispherical phased array with 1,000 transducer elements and MR temperature imaging of focal heating. Specifically, the system combines a 30 cm diameter hemispherical 1,000-element phased array transducer operating at 650 kHz with a 1,000-channel driving system, a treatment planning/MRI thermometry/dosimetry workstation, and a water cooling/circulation/degassing system that is integrated with a clinical 3T MRI unit (GE Medical Systems, Milwaukee, WI) to allow for individual control of the phase and amplitude for each element in the phased array to electronically steer the beam and to correct for skull-induced beam aberrations. It is mechanically positioned initially at the center of the desired target, and steering around this point is achieved with the phased array. The first generation of this system has been successfully tested in patients at the Brigham and Women’s Hospital [51] to show that transcranial focusing is possible and safe (see Fig. 28.4), and it is currently undergoing clinical trials in Zurich, Switzerland, at the University of Virginia, and at Sunnybrook and Women’s Hospital in Toronto for functional neurosurgery. Thalamic lesions have been successfully created for the treatment of neurogenic pain [52] and essential tremor. Future planned applications will include Parkinson’s disease and epilepsy.
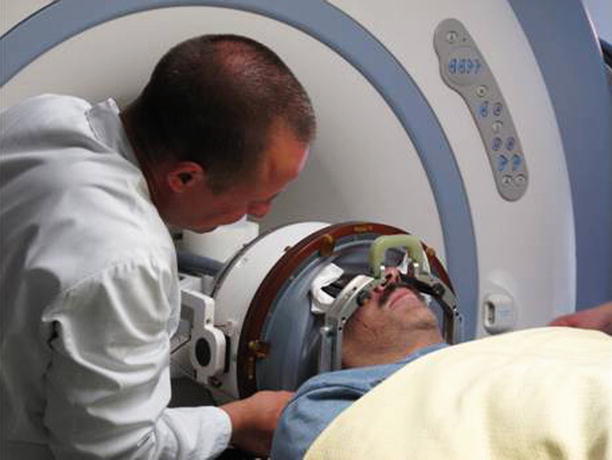
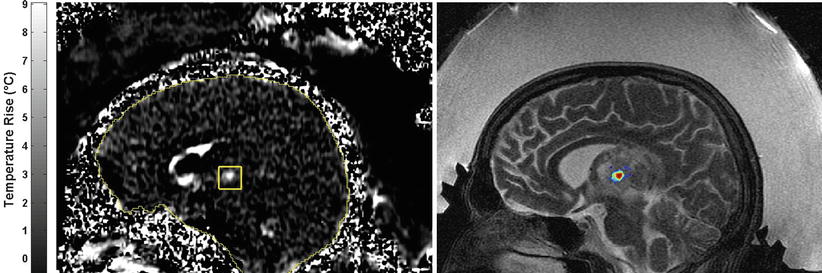
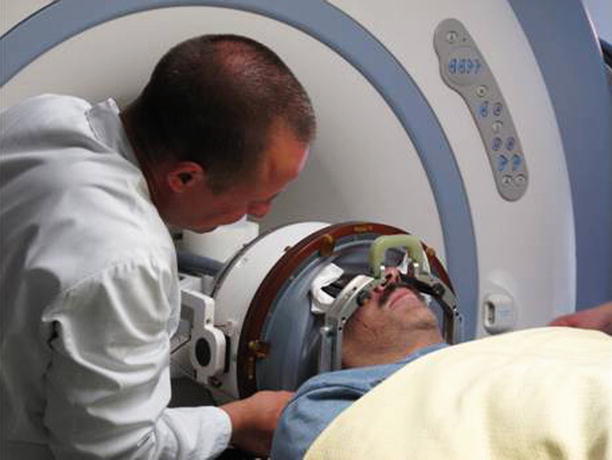
Fig. 28.3
The brain treatment system (ExAblate 4000, InSightec)
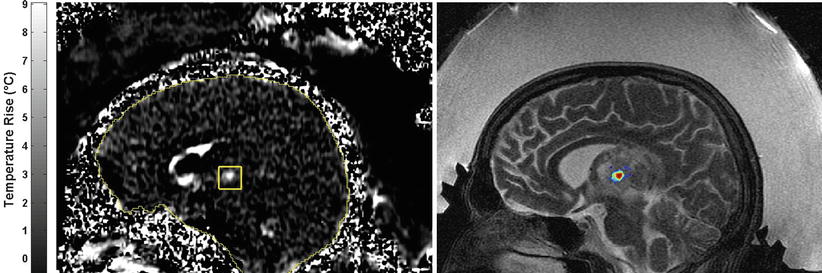
Fig. 28.4
Temperature-sensitive MRI showing the heating at the focal point within a thalamic tumor
The potential applications of MRgFUS are wide-ranging, and this technology holds tremendous potential for noninvasive lesioning of deep brain targets, overcoming trajectory constraints, minimizing tissue damage, and reducing the risks of invasive procedures. As with other applications of MRgFUS, delivering destructive energy deep in the brain without damaging any normal brain structure or function is consistent with the concept of “ideal surgery.” An additional benefit of using MRI guidance is for precision lesioning. The ability to provide MRI-targeted precision treatment without causing damage to any of the normal surrounding tissue offers many potential advantages. It is noninvasive, incisionless, and bloodless and without burr holes or penetrating electrodes, reducing the risks of hemorrhage and infections. Since the skull remains intact, there is no shifting of any tissues or neural structures within the brain. MRgFUS does not use ionizing radiation so treatment may be repeated and staged as disease progresses and has no risks of toxicity and accumulated dose effects.
Targeted Drug Delivery Through Transiently Opened BBB
The blood-brain barrier (BBB) normally serves to protect the brain from agents that circulate in the blood. However, it also poses a major limitation to drug delivery and the large majority of drugs cannot be used in the central nervous system (CNS) [53]. Specifically, the BBB is a composite of endothelial structures that exclude over 98 % of small-molecule drugs and almost 100 % of large-molecule neurotherapeutics from being transmitted into the brain. Current methods to overcome the BBB are invasive and nontargeted and/or require the development of new drug carriers [54]. They include direct injection or the use of catheters and implantable devices to infuse substances into the brain. These invasive procedures increase the risk of infection and are not very efficient due to limited diffusion of the drugs into the brain. The BBB can be also reversibly disrupted by intra-carotid arterial infusion of hyperosmolar solutions and vasoactive agents. However, this method, the BBB disruption, is nontargeted and may have toxic side effects, including possible neuronal damage. Recent approaches for drug delivery include design of new drugs (or carriers) such as liposomes and nanoparticles or conjugation of the drugs with proteins, peptide vectors, or antibodies and use of endogenous BBB carrier systems to ferry drug molecules through the BBB. While these carriers can be effective, they may be limited because only a finite amount of drug molecules can be delivered due to a limited number of receptors and a discrete quantity of molecules attached to the carriers. They can also deliver to too many areas of the brain or everywhere in the brain, which may not be necessary or desirable. Therefore, the presence of the BBB places enormous restrictions on what drugs can be used in the CNS and drastically limits drug development. This limitation is reflected in the market for CNS drugs, which based on need should be equal to or greater than the market for other diseases. As many as one third of the population is expected to experience a CNS disorder in its lifetime, but the global pharmaceutical market for CNS-related drugs would have to increase by a factor of five to equal that for cardiovascular disease. Again, the approaches described above to circumvent the barrier are invasive and nontargeted or require a huge expense of developing complex new drug systems, making the opportunity that FUS technology represents in terms of overcoming these restrictions important and possibly necessary.
Short, low-intensity FUS bursts combined with a microbubble-based ultrasound contrast agent can result in targeted, temporary BBB disruption [55] that is safe and can even allow for the delivery of large-molecule agents to the brain. This technique potentially could represent a fundamental change in the treatment of CNS disease, creating new opportunities for drug development and allowing new uses of currently available drug therapies. With this technology combined with MR imaging, one could develop truly image-guided drug delivery and achieve a drug concentration precisely tailored to an individual patient’s CNS pathology.
With the combination of relatively low-intensity ultrasound bursts with a microbubble-based ultrasound contrast agent (USCA), we can reliably produce BBB disruption in a focal zone that lasts for a few hours and is associated with minor or no unwanted tissue effects [56, 57] (see Fig. 28.5). This technique takes advantage of the microbubbles’ response to ultrasound to mechanically stimulate and/or modify endothelial cells and the “tight junctions” in the brain. The presence of the microbubbles targets the ultrasound effects directly to the local microvasculature. The microbubbles in the USCA can interact with the ultrasound beam in several ways. At relatively low intensities, microbubbles oscillate within the ultrasound field to grow in size via rectified diffusion. This oscillation, along with radiation force on the bubble along the direction of the ultrasound beam, can apply force to the walls of micron-sized capillaries in the brain. In addition, near an oscillating bubble, the medium undergoes a small-scale micro-streaming, that can exert shear stresses on the endothelium. At high intensities, the bubbles can collapse violently, a phenomenon known as “inertial cavitation,” producing effects such as shock waves and high-velocity jets that result from inertial cavitation of the USCA microbubbles presumably produced by the extravasated erythrocytes observed when the exposure levels are too high. Without the USCA, the exposure levels used for BBB disruption are lower than the inertial cavitation threshold for tissue.
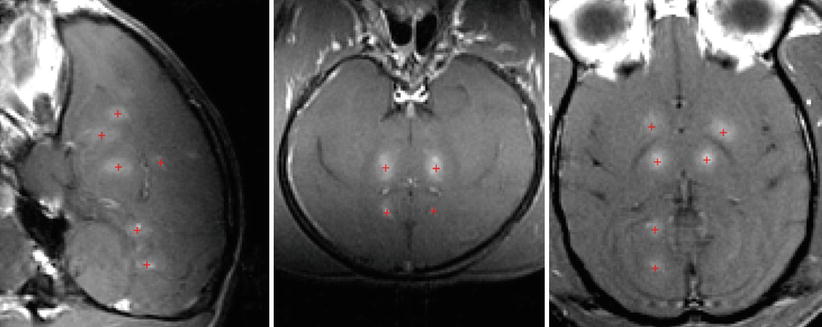
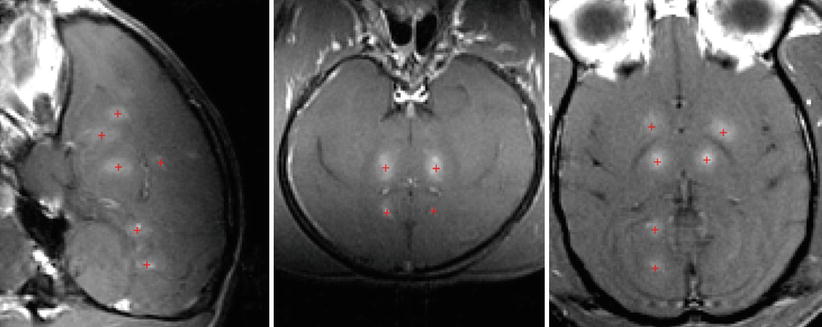
Fig. 28.5
Contrast‐enhanced MRI showing blood-brain-barrier disruption produced at different brain targets in a monkey. The focal point was steered to the different brain targets using the phased array. The + sign (red) represents the sites of open blood-brain barrier with contrast enhancement (Gadolinium-DPTA)
The goal is to apply exposures that elicit the mechanical stimulation (oscillation, radiation force, micro-streaming) on the vessel walls without inertial cavitation [58]. These effects appear to result in both active and passive transport mechanisms. Under electron microscopy, tracers have been observed to pass the BBB both between endothelial cells, suggesting that the exposures may be directly modifying the tight junctions, and across the endothelial cells through active vesicular shuttling, suggesting that the exposures can also trigger a cellular or vascular response [56].
The low-level exposures used for BBB disruption are expected to produce no or only negligible heating either at the focal point or in the skull. The MR temperature measurement acquired during the sonications confirms that no significant heating occurs. Precise targeting is achieved using MR temperature imaging to detect focal heating in the brain at low-energy, continuous-wave ultrasound exposures that produce temperatures below the thermal damage threshold that can be detected using MRI [46]. As an alternative, it is possible to use a modified MR elastography technique to map the focal displacements caused by radiation force during short ultrasound bursts [59] to localize the focal point during the sonications. This technique utilizes displacement-encoding gradients to map the displacement produced during each pulse. In our preliminary tests, we were able to detect displacements on the order of a few microns.
A wide range of molecular sizes, including an antibody-based anticancer agent, can pass through the BBB disruption. Also, therapeutic concentration of a chemotherapy agent can be delivered [60, 61], and that technique can provide a therapeutic response in inoculated tumors [62]. Enabling in the brain the full arsenal of available drugs and removing limitations that currently hinder drug development for CNS disease would obviously lead to long-term improvements and growth in research, public health, and health-care delivery. In addition to cancer therapies, this technique has potential application for most CNS disorders and for delivering diagnostic agents for advanced imaging. Whereas most brain disorders may benefit from this approach, the first obvious target for this technology would be to deliver anticancer drugs to brain tumors and the surrounding brain. While tumor blood vessels often lack a BBB, infiltrating cells at the margins are protected by a normal BBB. Furthermore, there exist other hurdles to effective drug delivery in tumors even if its blood vessels are leaky, such as high interstitial pressures, which might be overcome if we can temporarily increase tumor vascular permeability. While primary brain tumors may be good targets for this technology, a more immediate target could be brain metastases from non-CNS tumors. In many cases, drugs have been found to be effective for primary and metastatic tumors in other parts of the body but fail for brain metastases. Having a method to safely and precisely produce temporary BBB disruption in desired brain regions would overcome these hurdles, drastically increasing the options available to researchers, drug developers, and patients. The ability not only to enable the delivery of agents to the brain noninvasively but also to tailor the delivery to only the desired target will be a powerful tool for clinicians and neuroscientists.
Other Applications
In addition to tissue ablation and targeted drug delivery, FUS has been investigated for a number of other therapies in the brain [63], including notably therapies for stroke (sonothrombolysis, enhanced delivery of thrombolytic agents) [64] and for neuromodulation [65–67]. Currently there are promising attempts to treat Alzheimer’s disease [68] to facilitate the crossing of stem cells through BBB [69] and applying FUS gene therapy [70, 71].
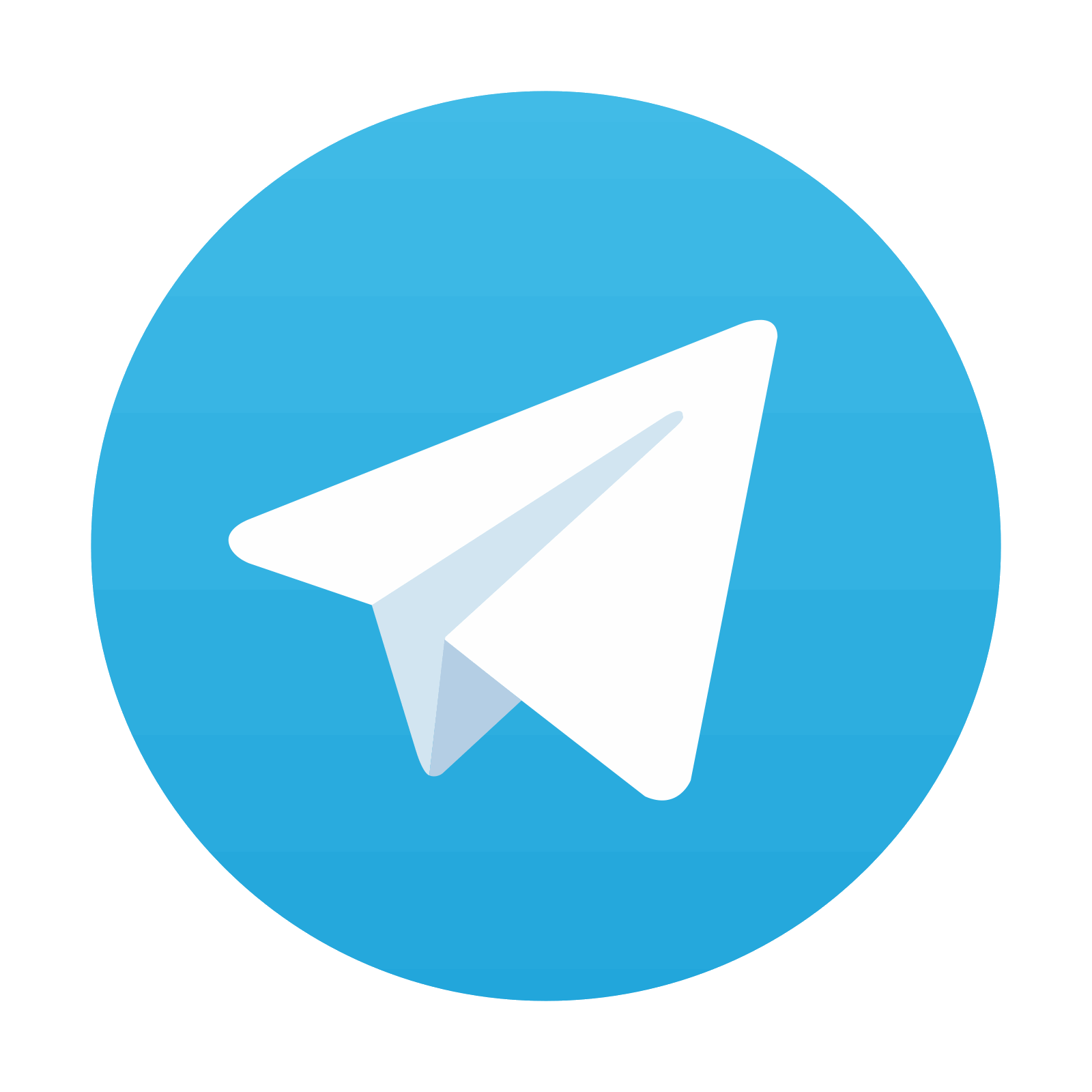
Stay updated, free articles. Join our Telegram channel
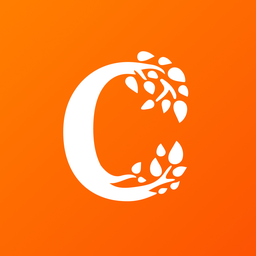
Full access? Get Clinical Tree
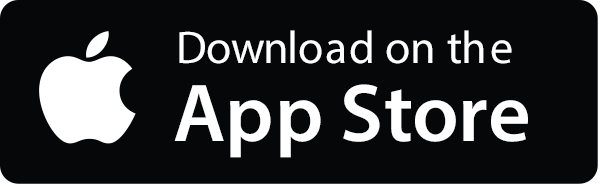
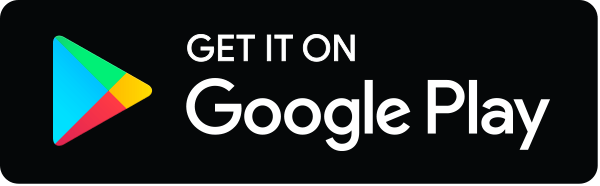