Neuroimaging of Traumatic Brain Injury
3.1 Introduction
Traumatic brain injury (TBI) is a major public health and socioeconomic problem in the United States and throughout the world. The U.S. Centers for Disease Control and Prevention estimates that approximately 1.7 million Americans sustain a TBI annually, resulting in 1.3 million visits to the emergency department, 275,000 hospitalizations, and more than 50,000 deaths related to TBI annually.1 Clearly, TBI is a leading cause of death among young adults in the United States. These numbers underestimate the actual magnitude of TBI because the statistics are unknown for those who do not seek medical care or who have no access to care. Moreover, long-term disability as a result of TBI is being increasingly recognized as a public health issue. It is estimated that at least 5.3 million Americans (up to 2% of the U.S. population) suffer from long-term or lifelong disability as a result of TBI.2
The common causes of TBI are falls, motor-vehicle crashes, bike accidents, sports-related injuries, assault and battery, and most recently blast injuries among soldiers returning from the Iraq and Afghanistan wars.3 In Seattle, a city of motorcycle and bicycle enthusiasts, accidents are frequent and occasionally fatal among youth, despite mandatory bicycle-helmet-use laws in King County and Washington State.4
The vast majority of sports-related TBIs are so-called mild traumatic brain injury (mTBI), or concussion.5 Concussion presents unique features of TBI in which emotional and behavioral psychological symptoms persist in some of the victims. Conventional imaging does not usually demonstrate abnormality, and advanced imaging has been tested with variable results. This topic is discussed in detail in Chapter ▶ 11 of this book.
Traditionally, The Glasgow Coma Scale (GCS) has classified TBI as mild (GCS 13–15), moderate (GCS 9–12), or severe (GCS 8 or lower). The GCS was developed in 1974 by Bryant Jennett, a neurosurgeon at the University of Glasgow.6 The GCS is a simple, reproducible clinical assessment tool of the state of consciousness of a patient and has been used widely in first aid, emergency medical services, and intensive care unit settings for the last 16 years.7,8 Three components of GCS score include eye response (4 points), verbal response (5 points), and motor response (6 points) (▶ Table 3.1). It is useful for estimating the prognosis for TBI patients; however, it does not provide mechanistic or pathophysiological information related to TBI. It is well known that clinical and imaging findings vary widely among patients classified for the same GCS score and that these patients often require different clinical management.9 Moreover, the accuracy of the GCS score is often reduced by early sedation and intubation of TBI patients.10
Eye opening (E) | |
Spontaneously | 4 |
To verbal stimuli | 3 |
To pain | 2 |
Never | I |
Best motor response (M) | |
Obeys commands | 6 |
Localizes pain | 5 |
Flexion withdrawal | 4 |
Flexion abnormal | 3 |
Extension abnormal | 2 |
No response | 1 |
Best verbal response (V) | |
Orientated and converses | 5 |
Disoriented and converses | 4 |
Inappropriate words | 3 |
Incomprehensible words | 2 |
No response | 1 |
One of the major downsides of GCS score is the limited utility of GCS for mTBI. GCS was not intended to address the level of injury or consciousness for patients with mTBI.
The Glasgow Outcome Scale (GOS), on the other hand, focuses on how head injury affects the functional status of life. Each patient is assigned to one of five possible outcomes: death, persistent vegetative state, severe disability, moderate disability, and good recovery. Extended GOS further divides the outcomes into eight categories (▶ Table 3.2).11
1 | Death | D |
2 | Vegetative state | VS |
3 | Lower severe disability | SD – |
4 | Upper severe disability | SD + |
5 | Lower moderate disability | MD – |
6 | Upper moderate disability | MD + |
7 | Lower good recovery | GR – |
8 | Upper good recovery | GR + |
Use of the structured interview is recommended to facilitate consistency in ratings. |
3.2 Imaging Workups for Traumatic Brain Injury
In the acute traumatic phase, computed tomography (CT) scan remains the first line of diagnostic imaging to evaluate the severity of TBI and the extent of the injury, which helps in triaging patients who need surgical intervention from those who can safely be observed clinically.12 Unconscious or obtunded patients who arrived in the emergency room need an immediate head CT to assess the presence of intracranial hemorrhage, cerebral edema, and/ or herniation. A large expanding hematoma or hematoma with active extravasation needs to be evacuated to control hemorrhage in a timely fashion. Normal head CT allows patients to be discharged from the ED unless clinical signs and symptoms suggest more serious injury.
The CT scan is also the best imaging modality to evaluate fractures in the skull or skull base. The location of such fractures potentially necessitates further imaging workup for vascular injury, such as traumatic dissection or venous thrombosis or occlusion. Chapter ▶ 7, Blunt Cerebrovascular Injury, covers this type of injury, and Chapter ▶ 9, Skull Base Trauma, covers the anatomy of the skull base and fractures therein. Chapter ▶ 10, Maxillofacial Trauma, and Chapter ▶ 11, Traumatic Orbital Injury, discuss these types of injuries in detail.
Little discussion has taken place as to which severe and moderate TBI patients require head CT at arrival: A question is, which patients with mTBI should receive head CT in the ED and which patients can safely avoid CT scan? A CT scan involves ionizing radiation. Even though brain is not a particularly radiosensitive organ, judicious use of head CT is essential, in particular, in the pediatric patient population. In addition, the cost associated with CT scan for minor head trauma should be weighed against the benefits of CT. Several prediction rules and guidelines have been developed and validated to address this question.13–15 The prediction rules for head CT indication for mTBI are covered extensively in Chapter ▶ 22, Evidence-Based Imaging and Prediction Rules. It was reported that approximately 10 to 35% of head CT scans performed for minor head trauma in an urban level I trauma center did not meet the guidelines for an mTBI.16
Another common clinical question is whether or not to repeat head CT for patients with TBI. In an era of cost containment and judicious use of CT for radiation concern, this is a relevant question with conflicting evidence in literature. Meta-analysis of mTBI patients revealed that the routine follow-up head CT is not indicated unless patients present with clinical deterioration.17 A prospective study enrolling all types of TBI patients addressing the impact of management by the repeat head CT between a “clinically indicated” group and “routine follow-up” without clinical deterioration showed that approximately 20% of patients who underwent repeat head CT for clinical deterioration resulted in changes in the case management; these changes were seen in more severely injured and younger patients. This study did not support the routine use of repeat head CT without clinical deterioration.18 However, a recent study on repeat CT for 360 mTBI patients with abnormality on the initial head CT reported that approximately 30% of patients had injury progression even though clinical symptoms and signs remained stable in the first 8 hours.19 Those patients had a higher injury severity score, intubation, and higher mortality rates. The presence of a mass effect on the initial CT was independently associated with worsening CT findings.
Despite its higher sensitivity in detecting TBI lesions, magnetic resonance imaging (MRI) of the brain is not routinely used clinically for acute TBI, and its routine use is not supported in the literature,20 in part because of relatively limited access to MRI and difficulty in monitoring patients with severe injury inside the scanner. Several studies reported that brain MRI showed more TBI lesions compared with the CT study at the time of the patient’s arrival,21 but the study also indicated that there was no significant impact on the management of patients with TBI in the acute trauma setting.22 It has been reported that for about 30% of patients with mTBI with a negative head CT, brain MRI shows evidence of TBI. Most CT-negative, but MR-positive brain injuries are axonal injuries [diffuse axonal injury (DAI) and traumatic axonal injury (TAI)].
The workshop organized by the National Institute of Neurological Disorders and Stroke, with support from the Brain Injury Association of America, the Defense and Veterans Brain Injury Center, and the National Institute of Disability and Rehabilitation Research, outlined the problems related to the current TBI classification based on GCS and stated that “more widespread use of acute MRI will be important to provide additional detail necessary for accurate pathoanatomic classification, particularly of the TAI/DAI spectrum. Efforts should be coordinated to identify and eliminate barriers to the implementation of acute MRI for TBI clinical trials and to standardize and validate MRI grading system.9
Various magnetic resonance (MR) sequences have been investigated, including T2-weighted images, fluid-attenuated inversion recovery (FLAIR), gradient echo (GRE) images, susceptibility-weighted images (SWI), diffusion-weighted images (DWI), diffusion tensor images (DTI), and MR spectroscopy. A study of 56 mTBI patients revealed that SWI showed the largest number of lesions, followed by GRE images, FLAIR, and T2-weighted images.23 Another study confirmed that SWI images revealed 30% more mTBI lesions compared with CT scans.24
Microhemorrhages, which are sometimes seen in TBI patients, may evolve over time. A study comparing 1.5T and 3T GRE images demonstrated 50% more lesions detected on 3 T compared with 1.5 T and a negative correlation between the number of lesions and the time interval from trauma in 1.5T study but not in the 3T study.25
3.3 Prediction of Clinical Outcomes
The degree of TBI can be better categorized based on the morphologic abnormality on CT scan. Attempts have been made to incorporate CT findings to predict the clinical outcomes of TBI patients. Maas et al developed the prognostic model based on 2269 moderate to severe TBI patients from multicenter clinical trials to predict the 6-month outcome using the information available at patient admission (i.e., the Marshall CT score). The model discriminated well in the development population [area under the receiver (AUR) operating characteristic curve (0.78–0.80)], as well as in the two external validity studies [area under the curve (AUC), 0.83–0.89] from Europe and North America. The CT findings used in the Marshall CT score include the presence or absence of a mass (hematoma) lesion, a midline shift of more than 5 mm, a compressed or absent cistern, and evacuated mass lesions. This evaluation was modified to develop the Rotterdam CT score,26 which includes compression of the basilar cistern, midline shift greater than 5 mm, traumatic subarachnoid or intraventricular hemorrhage, and the presence of different types of mass lesions (▶ Table 3.3). Using this simple CT-based Rotterdam score, 6-month mortality was predicted (AUC 0.77) more accurately than using the Marshall score (AUR 0.67).26
Prediction Value | Score |
Basal Cisterns | |
Normal | 0 |
Compressed | 1 |
Absent | 2 |
Midline Shift | |
No shift of shift <= 5mm | 0 |
Shift > 5mm | 1 |
Epidural mass lesion | |
Present | 0 |
Absent | 1 |
Intraventricular blood or tSAH | |
Absent | 0 |
Present | 1 |
Sun Score | +1 |
The scores range from 1 (mildest) to 6 (most severe). The actual mortality corresponding to the Rotterdam CT Score for the total score 1, 2, 3, 4, 5, and 6 is 0%, 6.8%, 16%, 26%, 53%, and 61%, respectively. Data from Maas AI, Hukkelhoven CW, Marshall LF, Steyerberg EW. Prediction of outcome in traumatic brain injury with computed tomographic characteristics: a comparison between the computed tomographic classification and combinations of computed tomographic predictors. Neurosurgery. 2005;57(6):1173–1182. |
A small study investigating the predictive values of CT, T2-weighted images, FLAIR, and SWI showed that the volume of T2 and FLAIR abnormality most consistently discriminates between good and poor outcomes groups as measured by Eye Opening (E)-GCS, whereas SWI was the most sensitive imaging study for showing parenchymal hemorrhage, although it failed to discriminate outcomes.27 More recently, a multicenter study enrolling 135 mTBI patients demonstrated that 27% of mTBI patients with negative CTs had abnormality on MRI. This Transforming Research and Clinical Knowledge (TRACK) TBI study revealed that subarachnoid hemorrhage (SAH) is a predictor for poor outcomes after adjusting for demographics, clinical presentation, and socioeconomic status. More than one contusion on MR [odds ratio (OR) = 4.5] and more than four foci of hemorrhagic TAI (OR = 3.2) are independent predictors for a poor prognosis after adjusting for head CT, demographics, and clinical and socioeconomic factors.28
Extensive research using advanced MRI has been ongoing to address which mTBI patients suffer from neuropsychological deficits and need to refrain from activity to avoid a second impact injury. The current state of mTBI imaging research is discussed in detail in Chapter ▶ 12.
3.4 Imaging Findings of Primary Traumatic Brain Injury Lesions
In this chapter, the pathoanatomic description of TBI was made in accordance with the Head Injury Reporting and Data System (HIRADS) in collaboration with the American College of Radiology (ACR) Head Injury Institute. The HIRADS was created to standardize the terminology used to describe the CT or MR findings of traumatic brain injury and to gather data for investigation of natural history, effectiveness of intervention, and prediction of clinical outcomes across various institutions in the country.
The pathoanatomic classifications of TBI described in this chapter are as follows:
Skull Fracture
Epidural Hematoma (EDH)
Subdural Hematoma (SDH)
Subarachnoid Hemorrhage (SAH)
Contusion
Intracerebral hemorrhage
Intraventricular hemorrhage
Diffuse axonal injury (DAI) and Traumatic axonal injury (TAI)
Brainstem injury
Pituitary/hypothalamic injury
Thalamus and Deep gray matter injury
3.4.1 Skull Fracture
A sign of skull fracture, such as scalp laceration or hematoma, is one of the indications for head CT. Skull radiography should not be performed because as it does not reveal any underlying brain injury. Limited sensitivity may lead to false assurance and is potentially dangerous. Lack of skull fracture does not exclude underling brain injury. One study showed that mTBI patients (GCS 14 or 15) of skull fractures had a fivefold higher risk of requiring neurosurgery compared with patients without skull fracture.29
Skull fracture is classified as either open or closed. Open fracture describes a fracture with an overlying skin defect, and may be frankly visible. The following are the types of skull fractures that commonly seen (▶ Fig. 3.1):
Fig. 3.1 Various skull fractures. (a) Linear fracture, (b) depressed fracture, (c) comminuted fracture, (d) diastatic fracture, (e) diastatic fracture involving the right occipitomastoid suture. Three-dimensional volumetric image provides an excellent overview of fracture configuration and alignment, which helps surgical planning.
Linear: A break from the outer to the inner table in a straight line or branching pattern without displacement of bone. Linear fracture itself has no significant clinical consequences unless it is associated with epidural hematoma (often associated with middle meningeal artery injury) or venous sinus injury or thrombosis (often transverse sinus).
Depressed: Usually results from blunt head injury by an object (e.g., rock, hammer, baseball bat, or assault). Depressed fractures are often accompanied by severe TBI and a high risk of increasing intracranial pressure. Compound depressed fracture exposes the cranial content to extracranial space, bearing the risk of infection and contamination. Depressed skull fractures are usually treated surgically to remove pressure on the brain from fragmented bone pieces.
Comminuted: Fracture into three or more pieces. Comminuted fractures are often associated with depressed fractures, where the pieces of fragments are displaced inward.
Diastatic: Fractures involving one or more sutures, resulting in widening of fractured suture rather than break of bone. Diastatic fractures are common in infants and young children (i.e., younger than 3 years) because sutures are not yet fused. When seen in adults, diastatic fractures commonly occur in the lambdoid sutures. One of the complications for children with skull fracture is leptomeningeal cyst, which is a cerebrospinal fluid (CSF) collection caused by a dural tear, resulting in diastasis of suture and development of cyst in between. It is called a growing skull fracture (▶ Fig. 3.1b).
Greenstick fractures: Incomplete fractures. Broken bones are not completely separated but rather bend or deform as if bending a greenstick. This is also more common in children.
The TBI lesions are classified into primary and secondary. Primary lesions refer to immediate parenchymal injury occuring at the time of injury. The primary injury includes EDH, SDH, SAH/intraventricular hemorrhage (IVH), TAI/DAI, contusions, and hematoma. Direct injury to the cerebral vasculature is also a type of primary lesion. Secondary lesions are potentially avoidable brain damages that occur at variable time after injury. The secondary injury include, but not limited to, cerebral swelling (edema), brain herniation, hydrocephalus, ischemia, infarction, CSF leak, and encephalomalacia. A brainstem injury can be a primary or secondary injury (discussed later).
3.4.2 Epidural Hematoma
An EDH is a collection of blood between the inner table of the skull and dura matter (▶ Fig. 3.2). In normal circumstances, no epidural space is present because of the firm attachment of the outer dural layer to the inner table of skull. EDH is often associated with injury to the middle meningeal artery and its branches and thus is most common in the temporoparietal location (75%) (▶ Fig. 3.3).
Fig. 3.2 Epidural hematoma. (a) Illustration demonstrating the epidural space between inner table of calvarium and dural matter. (b) Right temporal skull fracture with biconvex epidural hematoma. (c) Right temporoparietal epidural hematoma (red arrow).
Fig. 3.3 Venous epidural hematoma. (a) T1-weighted sagittal image shows vertex venous epidural hematoma displacing the superior sagittal sinus inferiorly (short arrow). (b) Coronal Gradient echo image shows vertex venous epidural hematoma crossing midline over sagittal suture but does not cross the coronal suture (long arrow). (c) Coronal FLAIR image shows no underling brain injury.
The expanding hematoma strips dura from the skull but is usually confined to the epidural space and does not cross the suture line (coronal or lambdoid suture) in adults. EDH often, but not always, has a biconvex shape. Venous EDH, on the other hand, results from bleeding from the meningeal or diploic veins or the dural sinuses. Venous EDH, although rare, is most commonly seen in the vertex, posterior fossa, or anterior aspect of the middle cranial fossa (as a result of bleeding from the sphenoparietal sinus). EDH from the sphenoparietal sinus in the anterior middle cranial fossa is usually limited by the sphenotemporal suture laterally and orbital suture medially; it has a benign natural history and rarely requires surgical intervention.30 Although EDH usually does not cross the suture, that is not the case for vertex venous EDH; near the vertex, periosteum forms the outer layer of the superior sagittal sinus, and it is thus less firmly attached to the sagittal suture, often crossing the midline (The Fundamentals of Diagnostic Radiology by Brant and Helms54 (head injury classification) (▶ Fig. 3.3).
Acute EDH is generally hyperdense, although EDH containing a hypodense area represents unclotted blood, indicating an actively extravasating unclotted blood within the clotted hyperdense blood (the Swirl sign). This is an ominous sign in the acute trauma setting (▶ Fig. 3.4).31
Fig. 3.4 Epidural hemorrhage (EDH) with active extravasation. (a) Active extravasation of EDH with Swirl sign containing the area of hypodensity within the left temporal EDH. (b) Computed tomography performed post evacuation shows area of infarction in the left occipital lobe and thalamus resulting from compression of the left posterior cerebral artery.
Another unique feature of EDH is crossing the tentorium (▶ Fig. 3.5), unlike SDH. One of the common complications related to the posterior fossa venous EDH is venous sinus thrombosis or venous sinus injury. CT venogram is a quick and definitive imaging test to address this clinical situation (▶ Fig. 3.6).
Fig. 3.5 Venous epidural hemorrhage (EDH) in the posterior fossa. Venous EDH extending from supratentorial (a) to infratentorial space (b). This is clearly seen on coronal reformatted image (c).
Fig. 3.6 Epidural hematoma (EDH) in the posterior fossa with venous sinus thrombosis. (a) Right occipital fracture with posterior fossa EDH with punctate pneumocephalus. (b) Computed tomography venogram demonstrates displacement of right transverse sinus. (c) Slightly inferiorly, intraluminal thrombus is present (long arrow). (d) Sagittal reformatted image shows extension of thrombus into the jugular vein (short arrows).
Often EDH is associated with more favorable outcomes compared with subdural hematoma or subarachnoid hemorrhage. As seen in the Rotterdam Score (▶ Table 3.3), the presence of EDH lowers the score compared with the score when EDH is not present.
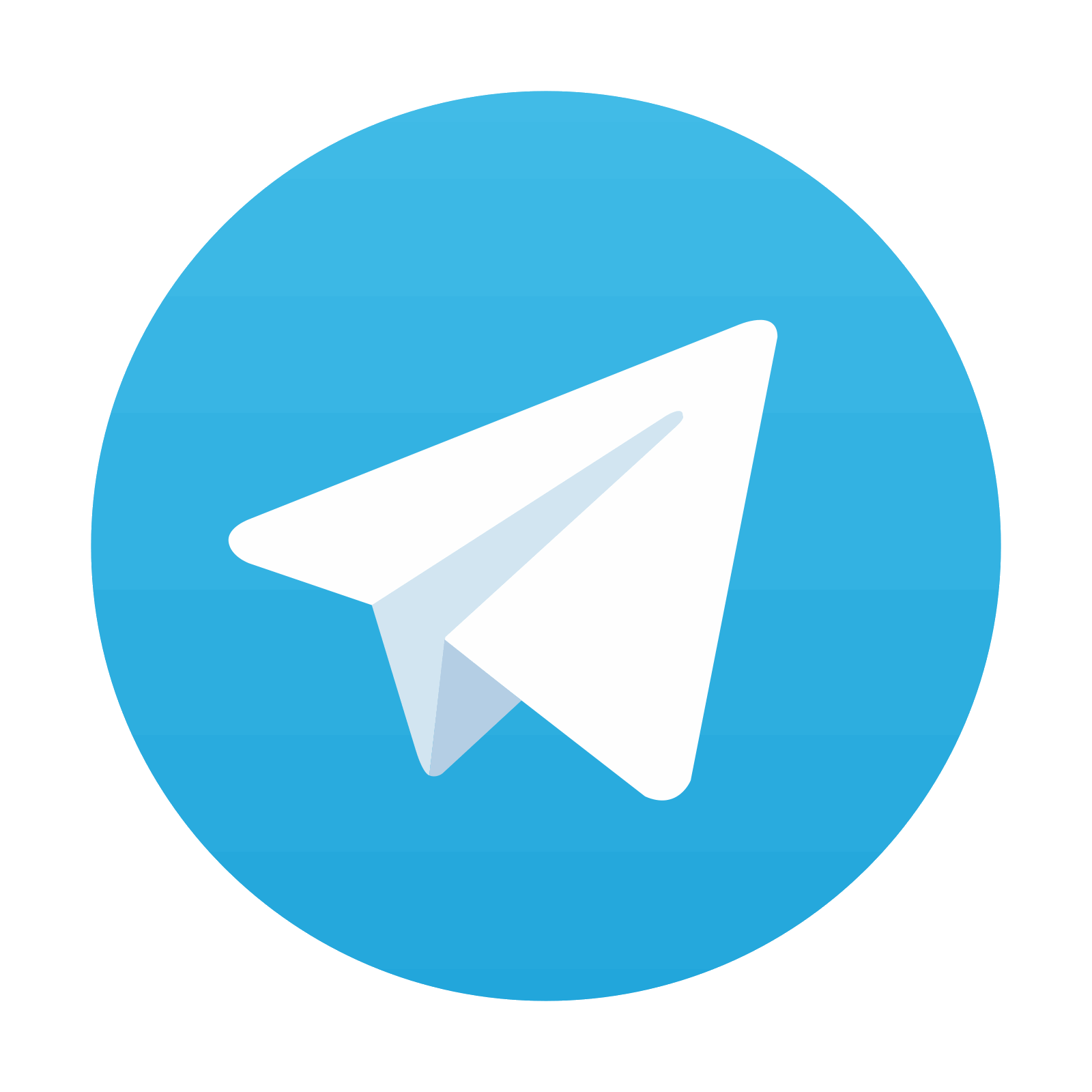
Stay updated, free articles. Join our Telegram channel
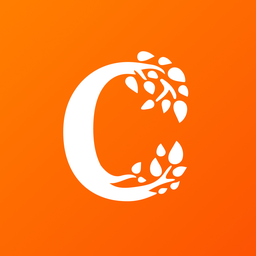
Full access? Get Clinical Tree
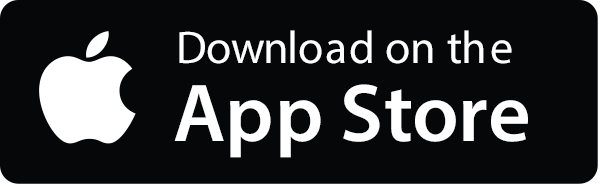
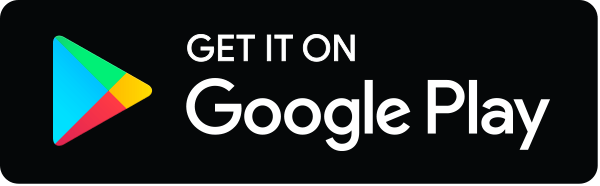