Fig. 12.1
Beam configuration for an intensity-modulated radiation therapy plan to treat a patient with non-small lung cancer. The beams are separated by 25–30° so as to avoid parallel opposed beams
Normal tissue constraints for radiation therapy used to treat thoracic malignancies are summarized in Table 12.1. At the authors’ institution, we attempt to minimize the total lung volume that receives >5 Gy (i.e., the V 5) to the greatest extent possible; we further restrict the mean lung dose (MLD) to 20 Gy or less and the volume of lung that receives >20 Gy (V 20) to <40 %. Other dose constraints include minimizing the mean dose and V 50 to the esophagus, restricting the cardiac V 30 to <45 %, and limiting the dose to the spinal cord to <45 Gy. We closely follow these and other dose-volume constraints based on summary QUANTEC recommendations for standard fractionation treatment.
Table 12.1
Dose-volume constraints for normal tissues during standard fractionation radiation therapy
Radiation only | Radiation with chemotherapy | Radiation with chemotherapy before surgery | |
---|---|---|---|
Spinal cord | D max <45 Gy | D max <45 Gy | D max <45 Gy |
Lung | MLD ≤20 Gy | MLD ≤ 20 Gy | MLD ≤20 Gy |
V 20 ≤ 40 % | V 20 ≤ 35 % | V 20 ≤ 30 % | |
V 10 ≤ 45 % | V 10 ≤ 40 % | ||
V 5 ≤ 65 % | V 5 ≤ 55 % | ||
Heart | V 30 ≤ 45 % | V 30 ≤ 45 % | V 30 ≤ 45 % |
Mean dose <26 Gy | Mean dose <26 Gy | Mean dose <26 Gy | |
Esophagus | D max ≤80 Gy | D max ≤80 Gy | D max ≤80 Gy |
V 70 < 20 % | V 70 < 20 % | V 70 < 20 % | |
V 50 < 50 % | V 50 < 40 % | V 50 < 40 % | |
Mean dose <34 Gy | Mean dose <34 Gy | Mean dose <34 Gy | |
Kidney | 20 Gy <32 % of bilateral kidney | 20 Gy <32 % of bilateral kidney | 20 Gy <32 % of bilateral kidney |
Liver | V 30 ≤ 40 % | V 30 ≤ 40 % | V 30 ≤ 40 % |
Mean dose <30 Gy | Mean dose <30 Gy | Mean dose <30 Gy |
12.2.2 Radiation Dose
Principles of basic radiobiology suggest that doses of 80–100 Gy are required to sterilize lung cancer [2]. The Radiation Therapy Oncology Group (RTOG) and other institutions have conducted randomized trials evaluating radiation doses of 60 Gy or more in combination with chemotherapy to treat inoperable NSCLC [3–7]. The optimal dose for definitive radiation therapy for patients with inoperable NSCLC at diagnosis is still controversial. At MD Anderson Cancer Center, such patients are treated with definitive radiation doses of 60–74 Gy with concurrent chemotherapy, if they can tolerate this therapy.
12.2.3 Radiation-Induced Toxicity
12.2.3.1 Radiation Pneumonitis
Patients receiving radiation therapy to the thorax are at risk for developing radiation pneumonitis (RP), which typically manifests within 3–9 months after the completion of radiation therapy. Many studies have demonstrated that MLD [8–15] and the percentage of lung volume receiving more than some threshold dose [8, 11, 13, 16–18] can predict the development of RP; however, other studies have shown that some of these factors are not linked with RP [16, 19, 20], but rather that only a history of smoking [11, 17], chronic obstructive pulmonary disease [9], and receipt of induction chemotherapy with mitomycin [10] predict RP.
Variables typically used in the evaluation of lung dose and risk of RP include the volume of both lungs receiving more than a threshold dose (V dose), the MLD, the lung V 20, and normal tissue complication probabilities (NTCPs) given various combinations of dose-volume variables. We and others also evaluate the lung V 5.
In general, in radiation therapy for lung cancer, the total tolerable radiation dose depends on the volume irradiated. In a retrospective analysis, the MLD (Fig. 12.2) and relative V 5–V 65 in increments of 5 Gy were all found to be associated with the incidence of grade ≥3 RP according to the Common Terminology Criteria for Adverse Events v3.0. Investigators at MD Anderson [21] showed that V 5 was also a significant predictor of RP (Fig. 12.2); in that study, the 1-year incidence of grade ≥3 RP for patients with a relative V 5 ≤ 42 % was 3 % compared with 38 % for those with V 5 > 42 % (P = 0.001). This finding suggests that damage to the lung, which has functional subunits in parallel, may depend more on the volume irradiated than on the radiation dose. Gopal et al. similarly demonstrated that exposing normal lung to as little as 13 Gy led to a pronounced decrease in diffusion capacity for carbon monoxide (DLCO), and a loss of DLCO of >30 % was associated with grade ≥2 pulmonary symptoms (P = 0.003). Those investigators concluded that such a low threshold for deterioration of DLCO (13 Gy) indicates that it is better to treat a small amount of normal lung to a high dose rather than treating a large volume to a low dose [22]. Similarly, Yorke et al. reported that in patients with NSCLC treated with dose-escalated radiation therapy, the incidence of grade ≥3 pneumonitis correlated with MLD (P ≤ 0.05). The dose response as a function of mean dose to the total lung rises steeply, beginning at approximately 10 Gy [10]. In clinical practice, V 20 is often used as a surrogate to evaluate total dose to the lung in radiation treatment planning. Graham et al., in their analysis of V 20 for predicting RP, stratified patients into risk groups and found that the incidence of RP increased steeply when V 20 levels were 40 % or higher [16].
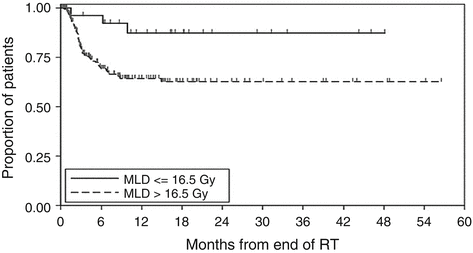
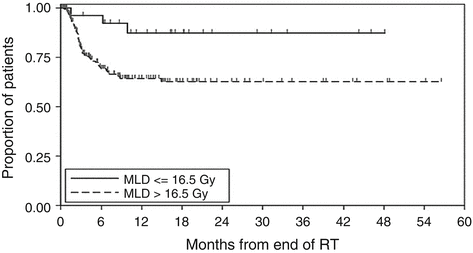
Fig. 12.2
Effect of mean lung dose (MLD) on freedom from grade ≥3 treatment-related pneumonitis. RT radiotherapy (Figure republished (with permission) from Wang et al. [21])
12.2.3.2 Esophagitis
Another form of radiation-induced toxicity, acute esophagitis, typically occurs within 90 days after the start of radiation therapy, whereas chronic esophagitis occurs after that time. Chronic esophagitis can result in the development of esophageal stricture requiring dilation and, in rare cases, esophageal fistula. Grade 1–2 radiation-related esophagitis is relatively common after treatment for lung cancer, and rates of grade >3 esophagitis range from 10 to 50 % [23–25]. A recent analysis of acute esophagitis in four RTOG trials involving 528 patients reported that 75 % of patients had grade >2 acute esophagitis and 34 % had grade >3 acute esophagitis after radiation therapy. Nineteen percent of these cases had developed within the first month of treatment, 32 % by the second month, and 33 % by the third month [26]. At the authors’ institution, we closely monitor acute esophagitis weekly during treatment, and we use aggressive supportive care measures to avoid the need for hospitalization and treatment interruptions.
Reports of potential clinical and dosimetric predictors of esophagitis are many, with substantial variation among studies. Esophagitis has generally been found to be associated with the volume of the esophagus receiving a specific dose, the mean esophageal dose, and the maximum esophageal dose (D max), having a history of esophageal morbidity, having nodal involvement, and receiving twice-daily rather than once-daily irradiation [27–32]. At the authors’ institution, we adhere closely to the following dose constraints for patients receiving high-dose radiation for thoracic malignancies: mean esophageal dose <34 Gy, V 70 < 20 %, and D max of 80 Gy. These dose constraints were based in part on the reported experience at Washington University [33]. A group at MD Anderson investigated the potential of IMRT for reducing the volumes of irradiated lung and esophagus during the treatment of NSCLC in a retrospective treatment planning study and found that IMRT produced lower lung V 20 and MLD than did three-dimensional conformal radiation therapy (3D CRT) in all cases. Notably, IMRT also led to smaller volumes of the esophagus and heart being exposed to radiation doses in excess of 45 Gy [34]. In a similar analysis, Gomez et al. tested the ability of a variety of factors to predict radiation-induced esophagitis in 652 patients with NSCLC treated with 3D CRT, IMRT, or proton beam therapy. In that study, the rate of grade ≥3 esophagitis was highest among patients who had been treated with IMRT (28 % vs. 8 % for 3D CRT and 6 % for proton therapy), leading the authors to conclude that the Lyman-Kutcher-Burman statistical model used in that study seriously underestimated the risk of severe esophagitis among patients treated with IMRT [35].
12.2.3.3 Cardiac Toxicity
Most of the posited effects of radiation-induced cardiotoxicity have been extrapolated from studies in which thoracic irradiation was delivered with older, 2D radiation techniques for breast cancer or lymphoma [36–40]. Findings from these studies, in which patients had been treated many years ago with techniques that could not minimize dose to the heart, are generally not applicable to current technology. Moreover, the reported rates of long-term cardiac morbidity varied considerably across studies, from <1 % to >15 %, although the rates do seem to continue to increase over time.
Several studies have compared the putative dosimetric advantages of IMRT over 3D CRT for sparing normal critical structures such as the heart. In one retrospective treatment planning comparison, Liu et al. investigated whether IMRT could reduce the volumes of lung and other critical structures relative to 3D CRT during radiation therapy for NSCLC. In addition to producing a lower MLD, IMRT led to smaller volumes of the esophagus and heart being exposed to high-dose radiation (>45 Gy). IMRT further allowed an additional safety margin around normal structures including the spinal cord, heart, and esophagus to account for uncertainties related to variations in setup, thereby minimizing the risk of radiation-associated cardiomyopathy [34]. Others at MD Anderson found similar results in their evaluations of IMRT versus 3D CRT for patients with stage III–IV NSCLC. Again, IMRT led to smaller lung V 10 and V 20 values as well as smaller MLD and a 10 % absolute reduction in risk of RP; IMRT also reduced the volumes of the heart and esophagus receiving >40–50 Gy. Those investigators concluded that IMRT could significantly improve target coverage and reduce the volume of normal lung irradiated to low doses; they further stated that the extent of low-dose exposure of normal tissues can be controlled in IMRT by choosing appropriate planning parameters [41].
12.3 Clinical Use of IMRT
As noted previously within this chapter, concurrent chemoradiation therapy is usually recommended for patients with locally advanced, inoperable stage IIIA or IIIB NSCLC. Nevertheless, treatment failures are relatively common, and overall survival rates remain relatively low at 5 years. Several studies have demonstrated that improving local disease control can improve overall survival for patients with stage III NSCLC [42–45]. Using IMRT rather than 3D CRT is thought to provide both dosimetric and clinical advantages when sufficiently high doses can be given for locally advanced NSCLC. IMRT enables tighter sculpting of high-dose regions around the target volume; the steep gradients created can reduce the radiation dose to surrounding normal tissues, which presumably could facilitate dose escalation [46].
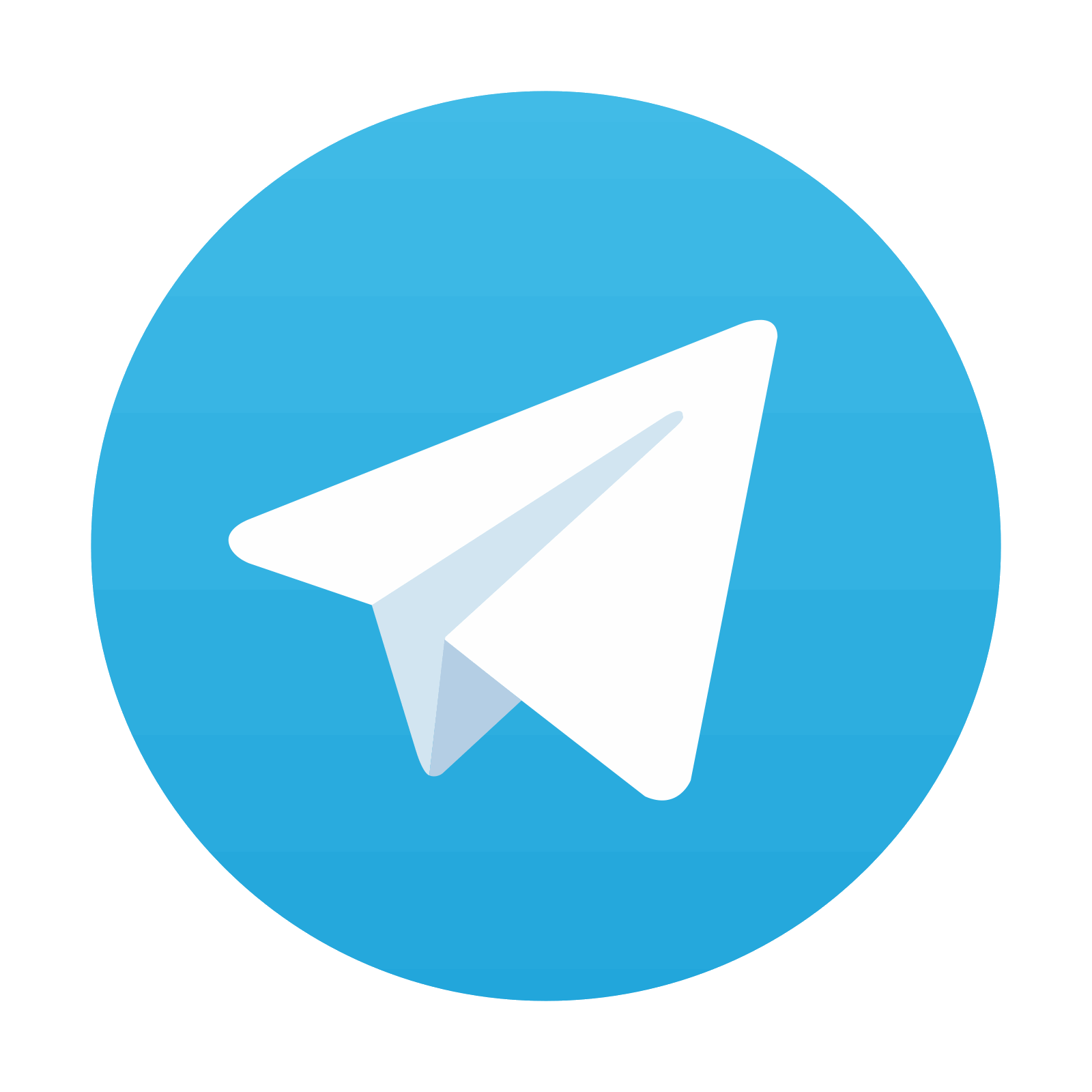
Stay updated, free articles. Join our Telegram channel
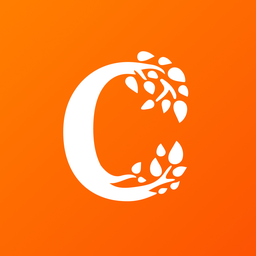
Full access? Get Clinical Tree
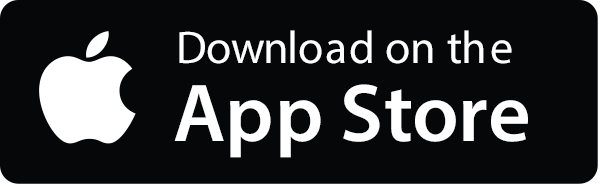
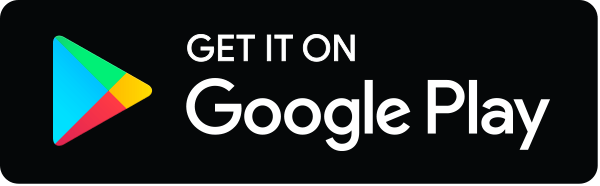