34 Nuclear medicine is a medical specialty that focuses on the use of radioactive materials called radiopharmaceuticals* for diagnosis, therapy, and medical research. In contrast to radiologic procedures, which determine the presence of disease based on structural appearance, nuclear medicine studies determine the cause of a medical problem based on organ or tissue function (physiology). Three important factors distinguish PET from all radiologic procedures and from other nuclear imaging procedures. First, the results of the data acquisition and analysis techniques yield an image related to a particular physiologic parameter such as blood flow or metabolism. The ensuing image is aptly called a functional or parametric image. Second, the images are created by the simultaneous detection of a pair of annihilation radiation photons that result from positron decay (Fig. 34-1). The third factor that distinguishes PET is the chemical and biologic form of the radiopharmaceutical. The radiotracer is specifically chosen for its similarity to naturally occurring biochemical constituents of the human body. Because extremely small amounts of the radiopharmaceutical are administered, equilibrium conditions within the body are not altered. If the radiopharmaceutical is a form of sugar, it behaves very much like the natural sugar used by the body. The kinetics or the movement of the radiotracer such as sugar within the body is followed by using the PET scanner to acquire many images that measure the distribution of the radioactivity concentration as a function of time. From this measurement, the local tissue metabolism of the sugar may be deduced by converting a temporal sequence of images into a single parametric image that indicates tissue glucose use or, more simply, tissue metabolism. PET is predominantly used to measure human cellular, organ, or system function. A parameter that characterizes a particular aspect of human physiology is determined from the measurement of the radioactivity emitted by a radiopharmaceutical in a given volume of tissue. In contrast, conventional radiography measures the structure, size, and position of organs or human anatomy by determining x-ray transmission through a given volume of tissue. X-ray attenuation by structures interposed between the x-ray source and the radiographic image receptor provides the contrast necessary to visualize an organ. CT creates cross-sectional images by computer reconstruction of multiple x-ray transmissions (see Chapter 31). The characteristics of PET and other imaging modalities are compared in Table 34-1. The differences between the various imaging modalities can be highlighted using a study of brain blood flow as an example. Without an intact circulatory system, an intravenously injected radiopharmaceutical cannot make its way into the brain for distribution throughout the brain’s capillary network ultimately diffusing into cells that are well perfused. For radiographic procedures such as CT, structures within the brain may be intact, but there may be impaired or limited blood flow to and through major vessels within the brain. Under these circumstances, the CT scan may appear almost normal despite reduced blood flow to the brain. If the circulatory system at the level of the capillaries is not intact, a PET scan can be performed, but no perfusion information is obtained because the radioactive water used to measure blood flow is not transported through the capillaries and diffused into the brain cells. An imaging technique that augments CT and PET is magnetic resonance imaging (MRI) (see Chapter 32). Images obtained with PET and MRI are shown in Fig. 34-2. MRI is used primarily to measure anatomy or morphology. In contrast to CT, which derives its greatest image contrast from varying tissue densities (bone from soft tissue), MRI better differentiates tissues by their proton content and the degree to which the protons are bound in lattice structures. The tightly bound protons of bone make it virtually transparent to MRI. CT, MRI, and other anatomic imaging modalities provide complementary information to nuclear medicine imaging and PET. These imaging modalities benefit from image coregistration with CT and MRI by pinpointing physiologic function from precise anatomic locations. Greater emphasis is being placed on multimodality image coregistration between PET, CT, SPECT, and MRI for brain research and for tumor localization throughout the body (Fig. 34-3). All new PET imaging systems are fused with a CT scanner for attenuation and anatomic positioning information. Newer SPECT imaging systems incorporate CT technology for the same purposes. The basic components of an atom include the nucleus, which is composed of varying numbers of protons and neutrons, and the orbiting electrons, which revolve around the nucleus in discrete energy levels. Protons have a positive electrical charge, electrons have a negative charge, and neutrons are electrically neutral. Protons and neutrons have masses nearly 2000 times the mass of the electron; the nucleus comprises most of the mass of an atom. The Bohr atomic model (Fig. 34-4) can describe this configuration. The total number of protons, neutrons, and electrons in an atom determines its characteristics, including its stability. To explain this process better, investigators have created decay schemes to show the details of how a parent nuclide decays to its daughter or ground state (Fig. 34-5, A). Decay schemes are unique for each radionuclide and identify the type of decay, the energy associated with each process, the probability of a particular decay process, and the rate of change into the ground state element, commonly known as the half-life (T½) of the radionuclide. Radioactive decay is considered a purely random and spontaneous process that can be mathematically defined by complex equations and represented by average decay rates. The term half-life is used to describe the time it takes for a quantity of a particular radionuclide to decay to one half of its original activity. This radioactive decay is a measure of the physical time it takes to reach one half of the original number of atoms through spontaneous disintegration. The rate of decay has an exponential function, which can be plotted on a linear scale (see Fig. 34-5, B). If plotted on a semilogarithmic scale, the decay rate would be represented as a straight line. Radionuclide half-lives range from milliseconds to years. The half-lives of most radionuclides used in nuclear medicine range from several hours to several days. The most commonly used radionuclide in nuclear medicine is 99mTc, which is produced in a generator system. This system makes available desirable short-lived radionuclides—the daughters—which are formed by the decay of relatively longer lived radionuclides—the parents. The generator system uses 99Mo (molybdenum-99) as the parent. 99Mo has a half-life of 66.7 hours and decays (86%) to a daughter product known as metastable 99mTc. Because 99mTc and 99Mo are chemically different, they can easily be separated through an ion-exchange column. 99mTc exhibits nearly ideal characteristics for use in nuclear medicine examinations, including a relatively short physical half-life of 6.04 hours and a high-yield (98.6%), 140-keV, low-energy, gamma photon (see Fig. 34-5 A,). The following characteristics are desirable in an imaging radiopharmaceutical: • Ease of production and ready availability • Lowest possible radiation dose to the patient • Primary photon energy between 100 keV and 400 keV • Physical half-life greater than the time required to prepare the material for injection • Effective half-life longer than the examination time • Suitable chemical forms for rapid localization • Different uptake in the structure to be detected than in the surrounding tissue • Low toxicity in the chemical form administered to the patient 99mTc can be bound to biologically active compounds or drugs to create a radiopharmaceutical that localizes in a specific organ system or structure when the radionuclide is administered intravenously or orally. A commonly used radiopharmaceutical is 99mTc tagged to a macroaggregated albumin (MAA). After intravenous injection, this substance follows the pathway of blood flow to the lungs, where it is distributed throughout and trapped in the small pulmonary capillaries (Fig. 34-6). Blood clots along the pathway prevent this radiopharmaceutical from distributing in the area beyond the clot. As a result, the image shows a void or clear area, often described as photopenia or a cold spot. More than 30 different radiopharmaceuticals are used in nuclear medicine (Table 34-2). Generally, the quantities of radioactive tracers used in nuclear medicine present no significant hazard. Nonetheless, care must be taken to reduce unnecessary exposure. The high concentrations or activities of the radionuclides used in a nuclear pharmacy necessitate the establishment of a designated preparation area that contains isolated ventilation, protective lead or glass shielding for vials and syringes, absorbent material, and gloves. The handling and administration of diagnostic doses to patients warrants the use of gloves and a lead syringe shield, which is especially effective for reduction of exposure to hands and fingers during patient injection, at all times (Fig. 34-7). Any radioactive material that is spilled continues to emit radiation and must be cleaned up and contained immediately. Because radioactive material that contacts the skin can be absorbed and may not be easily washed off, it is very important to wear protective gloves when handling radiopharmaceuticals. Scanners have evolved into complex imaging systems known today as gamma cameras (because they detect gamma rays). These cameras are still scintillation detectors that use a thallium-activated sodium iodide crystal to detect and transform radioactive emissions into light photons. Through a complex process, these light photons are amplified, and their locations are electronically recorded to produce an image that is displayed as a hard copy or on computer output systems. Scintillation cameras with single or multiple crystals are used today. The gamma camera has many components that work together to produce an image (Fig. 34-8). Computers have become an integral part of the nuclear medicine imaging system. Computer systems are used to acquire and process data from gamma cameras. They allow data to be collected over a specific time frame or to a specified number of counts; the data can be analyzed to determine functional changes occurring over time (Fig. 34-9, A and B). A common example is the renal study, in which the radiopharmaceutical that is administered is cleared by normally functioning kidneys in about 20 minutes. The computer can collect images of the kidney during this period and analyze the images to determine how effectively the kidneys clear the radiopharmaceutical (see Fig. 34-9, C to E). The computer also allows the operator to enhance a particular structure by adjusting the contrast and brightness of the image. Computers are necessary to acquire and process SPECT images (see next section). SPECT uses a scintillation camera that moves around the patient to obtain images from multiple angles for tomographic image reconstruction. SPECT studies are complex and, similar to MRI studies, require a great deal of computer processing to create images in transaxial, sagittal, or coronal planes. Rotating three-dimensional images can also be generated from SPECT data (Fig. 34-10, A). Computer networks are an integral part of the way a department communicates information within and among institutions. In a network, several or many computers are connected so that they all have access to the same files, programs, and printers. Networking allows the movement of image-based and text-based data to any computer or printer in the network. Networking improves the efficiency of a nuclear medicine department. A computer network can serve as a vital component, reducing the time expended on menial tasks while allowing retrieval and transfer of information. Consolidation of all reporting functions in one area eliminates the need for the nuclear medicine physician to travel between departments to read studies. Centralized archiving, printing, and retrieval of most image-based and non–image-based data have increased the efficiency of data analysis, reduced the cost of image hard copy, and permitted more sophisticated analysis of image data than would routinely be possible. Many nuclear medicine procedures require some form of quantitative analysis to provide physicians with numeric results based on and depicting organ function. Specialized software allows computers to collect, process, and analyze functional information obtained from nuclear medicine imaging systems. Cardiac ejection fraction is a common quantitation study (Fig. 34-11). In this dynamic study of the heart’s contractions and expansions, the computer accurately determines the ejection fraction, or the amount of blood pumped out of the left ventricle with each contraction. Nearly all camera systems used for whole-body imaging incorporate a dual-head design for simultaneous anterior and posterior acquisition. Whole-body imaging systems are used primarily for whole-body bone or whole-body tumor imaging and other clinical and research applications (Fig. 34-12). With SPECT, one to three gamma detectors may be used to produce tomographic images (Fig. 34-13). Tomographic systems are designed to allow the detector heads to rotate 360 degrees around a patient’s body to collect “projection” image data. The image data are reconstructed by a computer in several formats, including transaxial, sagittal, coronal, planar, and three-dimensional representations. The computer-generated images allow for the display of thin slices through different planes of an organ or structure, helping to identify small abnormalities. The most common uses of SPECT include cardiac perfusion, brain, liver (see Fig. 34-10, B), tumor, and bone studies. An example of a SPECT study is the myocardial perfusion thallium study, which is used to identify perfusion defects in the left ventricular wall. 201Tl is injected intravenously while the patient is being physically stressed on a treadmill or is being infused with a vasodilator. The radiopharmaceutical distributes in the heart muscle in the same fashion as blood flowing to the tissue. An initial set of images is acquired immediately after the stress test. A second set is obtained several hours later when the patient is rested (when the 201Tl has redistributed to viable tissue) to determine whether any blood perfusion defects that were seen on the initial images have resolved. By comparing the two image sets, the physician may be able to tell whether the patient has damaged heart tissue resulting from a myocardial infarction or myocardial ischemia (Fig. 34-14). A blending of imaging function and form is available. By merging the functional imaging of SPECT with the anatomic landmarks of CT, more powerful diagnostic information is obtainable (Fig. 34-15). This combination has a significant impact on diagnosing and staging malignant disease and on identifying and localizing metastases. This new technology can be used for anatomic localization and attenuation correction. According to manufacturers, statistics show that adding CT (for attenuation correction and anatomic definition) changes the patient course of treatment 25% to 30% from what would have been done when using the functional image alone. Principle: It is unclear how 99mTc-labeled diphosphonates are incorporated into bone at the molecular level; however, regional blood flow, osteoblastic activity, and extraction efficiency seem to be the major factors that influence the uptake. In areas in which osteoblastic activity is increased, active hydroxyapatite crystals with large surface areas seem to be the most suitable sites for uptake of the diphosphonate portion of the radiopharmaceutical. Radiopharmaceutical: The adult dose of 20 mCi (740 MBq) of 99mTc hydroxymethylene diphosphonate (HDP) or 20 mCi (740 MBq) of 99mTc methylene diphosphonate (MDP) is injected intravenously. The pediatric dose is adjusted according to the patient’s weight.
NUCLEAR MEDICINE
Principles of Nuclear Medicine
Comparison with Other Modalities
Physical Principles of Nuclear Medicine
BASIC NUCLEAR PHYSICS
NUCLEAR PHARMACY
Radiation Safety in Nuclear Medicine
Instrumentation in Nuclear Medicine
COMPUTERS
QUANTITATIVE ANALYSIS
Imaging Methods
WHOLE-BODY IMAGING
SPECT IMAGING
COMBINED SPECT AND COMPUTED TOMOGRAPHY IMAGING
Clinical Nuclear Medicine
BONE SCINTIGRAPHY
Bone scan
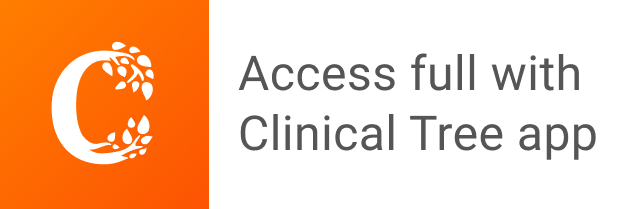