Fig. 2.1
Still frame of an angiogram showing a left anterior descending artery and its septal branches. (a) Flow is seen in the septal branches which course through the myocardium demonstrated by the red arrows. The tracing at the bottom of the picture demonstrates this is occurring during diastole. (b) Different frame from the same angiogram now shows no flow in the same small arteries during systole
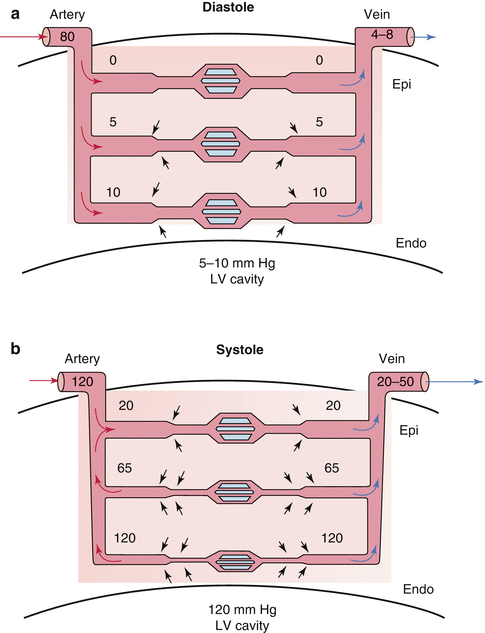
Fig. 2.2
Myocardial compression effects on transmural perfusion. (a) Compressive effects during diastole decrease in going from subendocardium to epicardium. At significantly elevated ventricular diastolic pressures of >20 and low diastolic aortic pressures of <60, the subendocardial region is at risk for ischemia during diastole. (b) During systole, ventricular contraction increases intramyocardial tissue pressure which cancels the arteriolar systolic pressure. Compression of venules increases venous outflow (From Mann et al. [2] and McCormack et al. [25] with permission)
In diastole, normal ventricular pressure is less than 10 mmHg and does not impede flow in the small arteries and arterioles which are coursing through the myocardium and have a pressure equal to that of aortic diastolic pressure. Thus, the perfusion pressure in the coronary arteries can be assumed to be the diastolic arterial pressure. Diastolic myocardial compressive effects on coronary perfusion, however, become significant when ventricular diastolic pressure rises as in decompensated heart failure, restrictive cardiomyopathy, or hypertrophic cardiomyopathy. These conditions may result in ventricular diastolic pressures of up to 40 mmHg. These diastolic compressive effects are also most prominent in the subendocardium making this area most prone to ischemia.
Coronary Artery Resistance
Coronary arterial flow (Q) is directly proportional to the perfusion pressure of the coronary arteries (P) and inversely proportional to the coronary vascular resistance (R).
As noted earlier, the coronary perfusion pressure is equal to the diastolic arterial pressure. Conditions such as hypotension or aortic regurgitation can decrease perfusion pressure by lowering diastolic pressure which leads to a decrease in oxygen supply. In normal conditions however aortic diastolic pressure does not change in order to increase coronary perfusion. Even during strenuous physical exercise, aortic diastolic pressure does not change significantly whereas systolic arterial pressure may rise considerably. This is due to fluctuations in vascular resistance. Coronary vascular resistance, therefore, is the other determinant of coronary blood flow and is inversely proportional to flow.

Blood flow through any artery is directly proportional to the fourth power of the vessel radius according to Poiseullie’s law, where r is the vessel radius, ΔP is the pressure difference between the two points, η is the blood viscosity, and L is the vessel length.
Therefore, the resistance of a vessel is directly proportional to the vessel length and inversely proportional to the fourth power of the vessel radius.
As we can see, given that the radius is raised to the fourth power, its importance in determining resistance and blood flow is essential. A 50 % decrease in the vessel radius will increase the resistance in that vessel by 16 times. This becomes important in determining the resistance of the epicardial coronary arteries which are prone to atherosclerosis (Fig. 2.3) [3]. Resistance also relates to the length of the vessel. This however is only a linear relationship whereas the radius is an exponential relationship. Furthermore, the length of the vessel cannot be changed physiologically but the radius can change through different mediators that will be explained below. As a result, the most regulated variable for control of coronary blood flow is vessel radius.
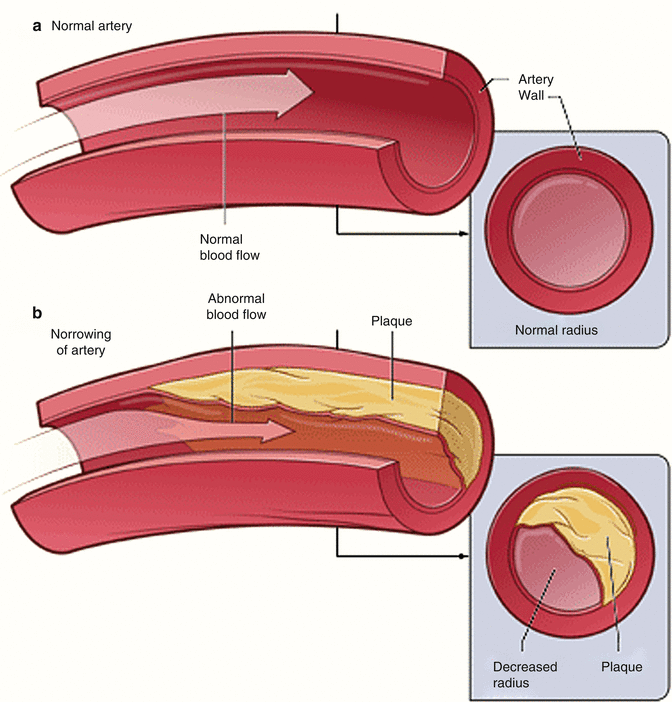

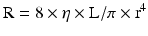
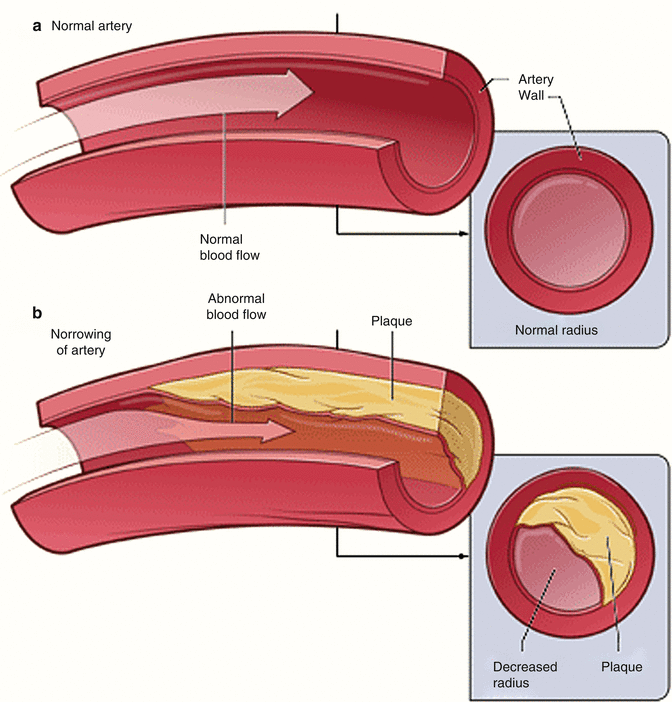
Fig. 2.3
Example of epicardial coronary artery with normal vessel radius (a). And decreased vessel radius due atheroslerosis (b) (Modified from National Heart, Lung, and Blood Institute; National Institutes of Health; U.S. Department of Health and Human Services)
Similarly to other systems, in the coronary circulation, the greatest resistance is at the arteriolar bed and this is where we see the largest drop in pressure. There is a significant decrease in vessel diameter going from the epicardial arteries to arterioles where the vessels are mainly connected in series leading to a large increase in the resistance of each individual vessel and increase in the total resistance of the system (at the arteriolar bed) (Fig. 2.4) [4]. While going from arterioles to capillaries, there is only a small decrease in vessel radius but a high degree of branching, equivalent to a system connected in parallel. The total number of capillaries in a circulation bed, therefore, greatly exceeds the number or arterioles resulting in a high cross-sectional area and low total resistance. Finally, as a result of the pressure drop in the arteriolar bed, blood flow in the microcirculation is no longer pulsatile but continuous.
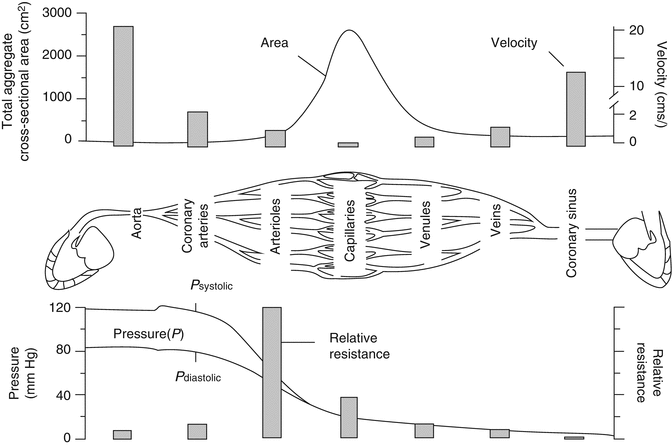
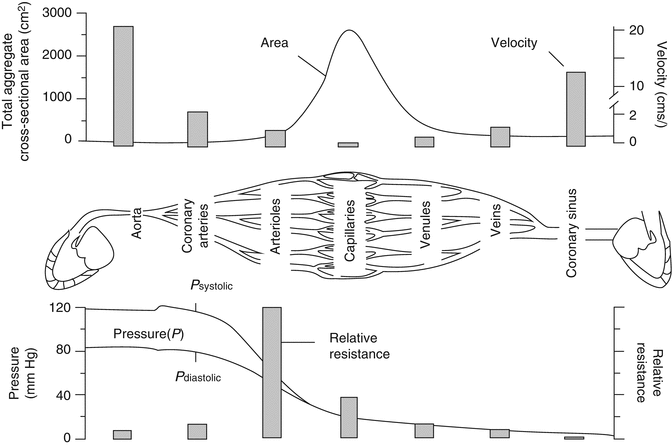
Fig. 2.4
Pressure, resistance, velocity, and flow in different parts of the coronary circulation (Modified from Stouffer [4] with permission)
Given that the greatest resistance is in the arteriolar bed then it is logical that the most regulation also happens in the arteriolar bed through constriction or dilation of coronary arterial vascular smooth muscle. Capillaries do not have smooth muscle therefore their resistance cannot be regulated that way.
Myocardial Oxygen Demand and Supply
In normal hearts, oxygen demand by the myocardium equals oxygen supply by the coronary arteries through autoregulatory mechanisms that are explained below [5] (Fig. 2.5). The three main determinants of myocardial oxygen demand are heart rate , contractility , and left ventricular wall stress. Increased heart rate leads to increased metabolic rate and higher oxygen demand while decreasing heart rate has the opposite effect. Contractility describes the force of contraction by the myocardium. This can be increased by sympathetic stimulation or positive inotropic medications such as dobutamine. Wall stress is the tension or stress that develops in the wall of the ventricle throughout the cardiac cycle and acts to pull the myocardial fibers apart. This stress during systole is called afterload and during diastole is termed preload. The wall stress is better described using the law of Laplace where σ is ventricular wall stress, P is ventricular pressure, r is ventricular radius, and h is ventricular wall thickness.
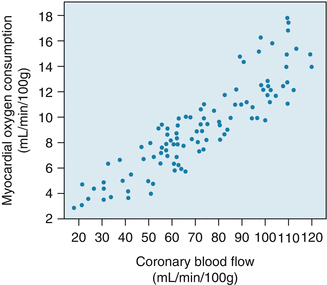
Wall stress, therefore, is directly proportional to ventricular wall pressure and radius. Disease states such as hypertension cause increased wall stress by increasing left ventricular (LV) wall pressure during systole, diastole, or throughout the cardiac cycle. This, in turn, leads to increased oxygen demand. Antihypertensive medications decrease LV wall stress thus decreasing oxygen demand. Other conditions that result in increased ventricular radius including dilated cardiomyopathy or valvular regurgitation will also cause an increase in wall stress and conversely an increase in oxygen demand. The third component of the equation (ventricular wall thickness) is inversely proportional to wall stress. In disease states such as chronic hypertension or aortic stenosis ventricular wall thickness will increase as a compensatory mechanism to attempt to decrease wall stress. Conditions that decrease wall thickness including myocardial infarction will result in increased wall stress and increased oxygen demand.
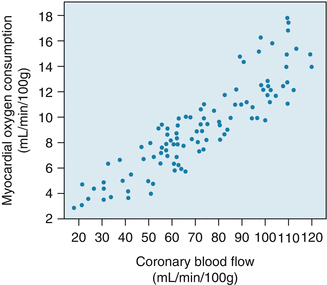
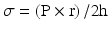
Oxygen delivery to the myocardium depends on coronary arterial oxygen content and coronary blood flow. Oxygen content is in turn determined by the hemoglobin concentration and degree of systemic oxygenation. In absence of anemia or lung disease, coronary arterial oxygen content remains fairly constant so increases in oxygen supply have to be met by increased coronary blood flow.
Furthermore, unlike other organs, oxygen extraction by the myocardium at rest is near maximum at about 65–75 % of arterial oxygen content. The heart at that point cannot simply increase oxygen extraction to increase oxygen supply to the myocardium. Instead, increased oxygen demand such as during exercise must be met by increasing blood flow to the myocardium.
Control of Coronary Flow and Autoregulation
The heart has the ability to maintain a constant blood flow at a range of coronary arterial pressures [6] (Fig. 2.6). Autoregulation of coronary vascular resistance makes this possible. Other organs that exhibit significant autoregulation are the brain and kidneys. Autoregulation helps the body to divert oxygen supply to organs (e.g. the heart) that need it the most in conditions when perfusion pressure may fall as in hypotension. Factors that participate in the regulation of coronary vascular resistance include local metabolites, endothelium derived substances, and neurohormonal innervation.
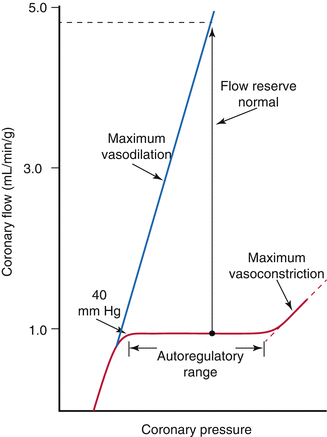
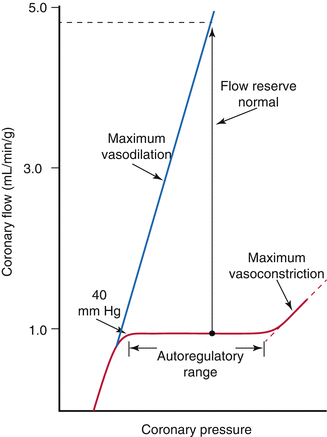
Fig. 2.6
Graph showing pressure-flow curve relationship in the coronary arteries. Coronary flow is maintained constant across a large range of perfusion pressures. The blue line represents maximal coronary flow also known as hyperemic flow (which will be discussed later on) (From Mann et al. [2] with permission)
Metabolic Factors
Local metabolic factors play the most important role in regulation of coronary blood flow. Some of these key local mediators include adenosine, ATP, oxygen, pH level, K+, and CO2. Myocardial metabolic activity parallels coronary blood flow and this is found in denervated hearts as well. Myocytes release vasodilatory substances in proportion to their level of work thus ensuring adequate supply to meet metabolic demands of the cells.
Adenosine is directly derived from hydrolysis of AMP (adenosine monophosphate) or from hydrolysis of adenine nucleotides. During oxygen supply/demand mismatch or increased metabolism, adenosine levels rise sharply as high energy phosphate bonds are consumed and ATP is converted to adenosine in cardiac myocytes or from adenine nucleotide hydrolysis. Adenosine then binds to A2 receptors on smooth muscle cells, increasing cyclic adenosine monophosphate (cAMP) which leads to vasodilation. At high levels, adenosine also activates endothelial adenosine triphosphate (ATP)-sensitive K+ (KATP) channels, vascular smooth muscle KATP channels, as well as calcium-activated potassium channels. These lead to decreased calcium entry into the vascular smooth muscle cells and vasodilation. During basal conditions, adenosine does not play a significant role in coronary vascular tone but the amount of adenosine generated during severe arterial hypoxia is sufficient to cause strong coronary vasodilation [7]. Conversely, when there is no mismatch in oxygen supply and demand, ATP levels are high and adenosine levels are much lower thus the amount of vasodilator substances is decreased.
Oxygen levels also affect regulation of coronary blood flow. Decrease of coronary arterial pO2 is one of the most powerful stimuli for coronary vasodilation. Coronary artery hypoxia can occur because of coronary artery stenosis, anemia, decreased ambient oxygenation like high altitude exposure, or blood flow impairment. Low myocardial tissue oxygen levels, whether resulting from reduction in O2 supply or increased demand, lead to opening of ATP sensitive K+ channels present in vascular smooth muscle cells. The latter then causes hyperpolarization of the cell membrane thus preventing Ca2+ channels from opening and leading to low cytosolic calcium levels [8]. This, in turn, causes smooth muscle relaxation and decreased vascular resistance.
Furthermore, during hypoxia, aerobic metabolism and oxidative phosphorylation are inhibited and ATP cannot be generated. Consequently ADP and AMP are converted to adenosine which also leads to vasodilation as mentioned above. In endothelial cells, hypoxia activates KATP channels that signal release of NO causing vascular smooth muscle relaxation as a result. This oxygen imbalance either from decrease in arterial oxygen content, decrease in coronary blood flow, or increase in cardiac metabolic rate decreases the oxygen supply/demand ratio and affects other vasodilator substances like pH and carbon dioxide levels. It should be stressed that a critical fall of coronary myocardial oxygenation likely does not occur in normal hearts under physiological conditions even during conditions of strenuous exercise. It may however be common if there is significant coronary stenosis.
Carbon dioxide is a byproduct of metabolism of pyruvate in the citric acid cycle. Increased levels of carbon dioxide will result in increased proton concentration and acidosis by the following equation
. Some experiments in animals have shown that increases in arterial carbon dioxide levels results in vasodilation and decreases in arterial carbon dioxide levels result in vasoconstriction [9]. However, its significance is not yet known in the autoregulation process.

Endothelial Factors
Vascular endothelial cells also produce vasoregulators that can act independently of cardiac metabolism to influence blood flow. Vasoactive substances produced by the endothelium include nitric oxide (NO), prostacyclin, endothelium derived hyperpolarizing factor (EDHF), and endothelin1 (Fig. 2.7) [10].
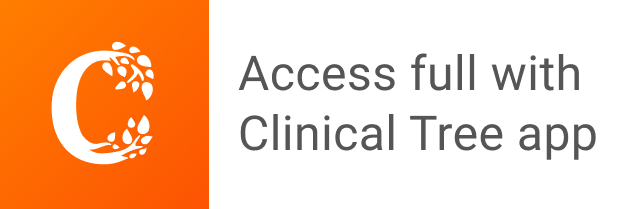