Fig. 1
Targeted and activatable probes are the kinds of agent used most widely in molecular imaging. Targeted probes bind via a ligand to their designated target, with the prototypical ligand being an antibody. Activatable agents are able to generate a signal only after interaction with their target, typically an enzyme, which typically activates the agent by cleavage. Because of their inherently greater complexity, the fabrication of these agents is also more difficult. Activated agents produce less background and result therefore in a better SNR
Molecular targeted therapy is getting increasingly important, especially in the form of so called “biologicals”, spearheaded by the success story of Gleevec, which targets a family of kinases involved in CML and also GIST tumors. Molecularly targeted agents are also used in Nuclear Medicine, for example as radiolabeled antibodies (Bexxar and Zevalin are clinically approved antibodies to treat lymphoma) or also small molecules such as 131I-MIBG for neuroblastoma therapy. These exemplify nicely the versatility of Molecular Imaging. A diagnostic scan with MIBG can evaluate if there is at all binding of the agent to the tumor and can also be helpful for dosimetry. If no sufficient binding occurs a molecularly targeted therapy with the same agent should be abandoned for this patient and other options sought. Molecular imaging is therefore not only being utilized to diagnose disease but is also at the forefront of personalized medicine, which seeks to tailor and monitor therapies to the biology of the individual. This allows one to first identify the presence of a suitable target, to initiate therapy with the same agent that has been used for imaging and subsequently to monitor this therapy over its course. Among the many benefits of this approach is the ability to detect failure of therapy early, and thus modulate treatment without loosing several weeks. Molecular Imaging is playing an ever-important role in this chain of events (Weissleder 1999, 2006; Rudin and Weissleder 2003).
Ironically, one of the major obstacles in the translation of molecular imaging agents into the clinic has been their specificity to a type or even subtype of disease, which limits their market value (Frangioni 2006). Given the tremendous regulatory efforts and costs associated with introducing an agent into the clinic, it is not surprising that successfull agents are those which are universally applicable—such as FDG, iodinated contrast agents, and Gadolinium chelates. Diseases with smaller incidence do not stand a chance in the current environment. The economic benefit (the return of investment) from a specific imaging agent is much too small compared with development costs. Unfortunately, there is currently (in the US) no orphan diagnostics regulation implemented, which would allow the development of useful agents also for smaller groups of patients with a devastating disease of lower incidence.
Designing targeted imaging probes can be challenging and has to consider several important points: (1) Identification of a suitable molecular target and with it recognizing the ligand best suited to bind with high specificity; (2) labeling the ligand with a marker for visualization geared toward the preferred imaging modality; (3) overcoming delivery barriers for the agent on its way to the target; and (4) exploiting strategies for signal amplification to increase sensitivity (Weissleder and Mahmood 2001; Grimm and Wunder 2005; Massoud and Gambhir 2003).
2 Identification of a Suitable Molecular Target
Identification of a suitable molecular target can be the most challenging part of the process to obtain a specific molecular imaging agent. Today, modern biotechnology has changed the odds somewhat as screening techniques have become more sophisticated and advanced. Technologies such as recombinant techniques, genomics and proteomics, robotics, and high-throughput screening have all contributed to the increased effectiveness of this aim. High-throughput screening is particular valuable as thousands of samples can be evaluated simultaneously and quantitatively (Kelly et al. 2009; Weissleder et al. 2005). We have shown that high-throughput screening can be performed both rapidly and reliably on many thousand samples utilizing a clinical MR scanner (Fig. 2) (Grimm et al. 2004; Högemann et al. 2002). Genomic screening has become widely available to enable discovery of suitable targets within tumors. For example, the gene expression profile of K-ras-activated lung tumors in a mouse model has been characterized, revealing a profile similar to that of human lung adenocarcinomas (Sweet-Cordero et al. 2005). The gene expression profile of K-ras tumors showed that several cathepsin proteases were strongly up-regulated in lung tumors compared with normal lung tissue. These proteases are also overexpressed in some patients with lung cancer, and their expression has been associated with tumor invasion and poor prognosis (Joyce 2004; Schweiger et al. 2000). We demonstrated subsequently that cathepsin activity could be used as a means of detecting these tumors with noninvasive in vivo optical imaging (Fig. 3) in the same mouse model (Grimm et al. 2005).
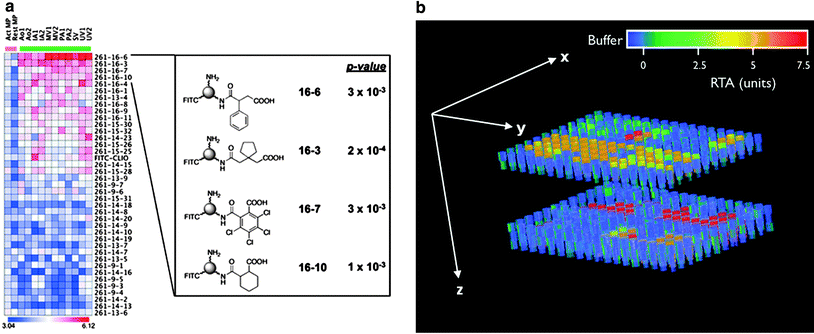
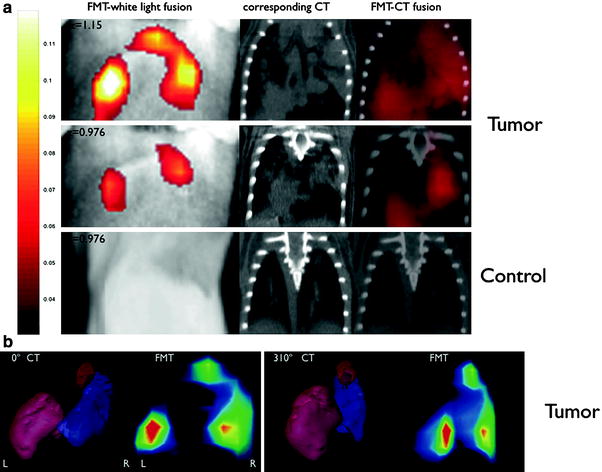
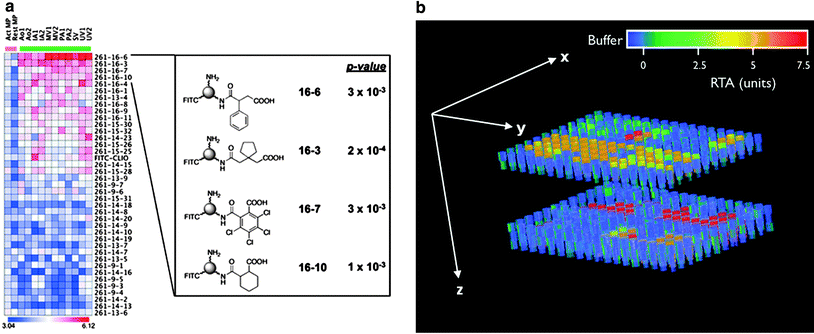
Fig. 2
a Binding of nanoparticles modified with a library of small molecules to macrophages (heat-map columns demarcated by pink bar) and endothelial cells (columns demarcated by green bar). The heat-map represents the log10 of bound nanoparticle concentrations (pM). Structures of four highly ranked small molecule-modified nanoparticles, along with their asymptotic permutation p-values, are shown at right. (Act MP and Rest MP activated and resting macrophages, respectively. Ao aorta, IA iliac artery, MV microvascular, PA pulmonary artery, SV saphenous vein, UV umbilical vein. FITC-CLIO = CLIO-NH2-FITC). Reprinted with permission from (Kelly et al. 2009). b High-throughput screening of telomerase activity utilizing a clinical 1.5 T magnetic resonance scanner. Data are shown as a three-dimensional (3D) reconstruction of T2 maps, derived from MR images of two 384-well plates stacked on each other and filled partly with 50 μl of buffer (blue), controls or telomerase containing samples; units on the color bar are given as relative units of telomerase activity. Reprinted with permission from (Grimm et al. 2004)
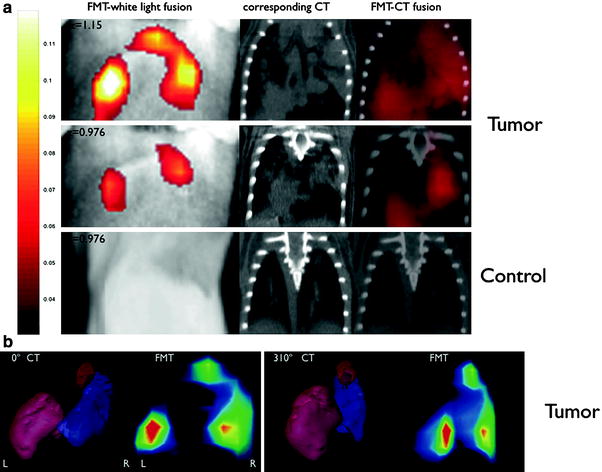
Fig. 3
Comparison of fluorescence-mediated tomography (FMT) and CT images of lung tumors in k-ras mice. FMT-white light fusion, CT, and FMT-CT fusion images of a a tumor-bearing mouse (two slices at different depths) and of a control mouse (one position). b 3D reconstructions of the bulk tumor mass in CT (Left) and of the FMT signal (Right) show a good correlation. Reprinted with permission from (Grimm et al. 2005)
3 Finding an Appropriate Ligand
Depending upon the method of target detection, a suitable ligand might be discovered at the same time, for example when a library of small molecules is used to screen for binding to the designated target. Small molecules can be easily coupled to nanoparticles as carriers and incubated with the target, which can be either cellular or molecular (or by computational modeling, in silico) (Grimm and Scheinberg 2011). The resulting best hit can than be pursued further for in vivo studies. Screenings like this have identified the amino acid glycine as a ligand, specifically binding to activated macrophages, which are often associated with tumors (Weissleder et al. 2005; Leimgruber et al. 2009). Another frequent approach is to identify a ligand peptide with phage display, with can be performed to achieve high specificity binding peptide sequences, in vitro or in vivo (Kelly et al. 2006). Once the most suitable ligand is determined based on the sensitivity, specificity and biodistribution of the ligand a label has to be attached (Weissleder and Mahmood 2001; Massoud and Gambhir 2003). For nuclear imaging, a variety of appropriate radioisotopes with different properties are available and already in clinical use. Especially promising is the novel PET tracer Zirconium-89 (89Zr). The unique physical decay properties and the energy of 89Zr (t 1/2 = 78.43 h, β+ = 22.3 %, E β+,max = 901 keV, E γ = 909 keV) are ideally suited for targeted imaging probes. The greater half-life provides copious activity at the required circulation times for optimal targeting to diseases sites (Holland et al. 2010a; Ruggiero et al. 2011). The longer half-life even enables imaging at later time points, including 120-h post injection, an imaging window difficult to achieve with the shorter lived PET nuclides 18F and 64Cu (Holland et al. 2010b, c). In MRI, paramagnetic metal ions such as chelated Lanthanides (Grimm et al. 2004; Aime et al. 2002; Montet et al. 2006; Tannous et al. 2006) or iron oxide nanoparticles (Grimm et al. 2004; Montet et al. 2006; Tannous et al. 2006) can be used for labeling. In optical imaging, organic fluorochromes or anorganic compounds such as quantum dots for fluorescence optical imaging are available but mostly exclusively for preclinical studies (Massoud and Gambhir 2003; Weissleder and Ntziachristos 2003), however, there was a recent study with the fluorochrome FITC targeted to folate receptor for intraoperative imaging (van Dam et al. 2011). Perfluorocarbon emulsion nanoparticles and acoustically reflective microbubbles are currently under investigation as ultrasound contrast agents (Dayton and Ferrara 2002; Schutt et al. 2003). For CT, various attempts have been made to develop targeted agents, however, the low sensitivity of CT is a considerable challenge. To obtain a decent contrast in CT with conventional agents, 300 mg/ml or more iodine needs to be present. Thus, considerable effort has been made to develop agents based on particles composed of elements with a high atomic number. These are mainly bismuth (Fig. 4) (Rabin et al. 2006) and gold nanoparticles (Kattumuri et al. 2007), which are particularly interesting as they can also be used for optical approaches, including Raman imaging (Zavaleta et al. 2009; Mu et al. 2010), which has also been proposed for intraoperative imaging (Kircher et al. 2012), however, none of these particles are so far clinically approved.
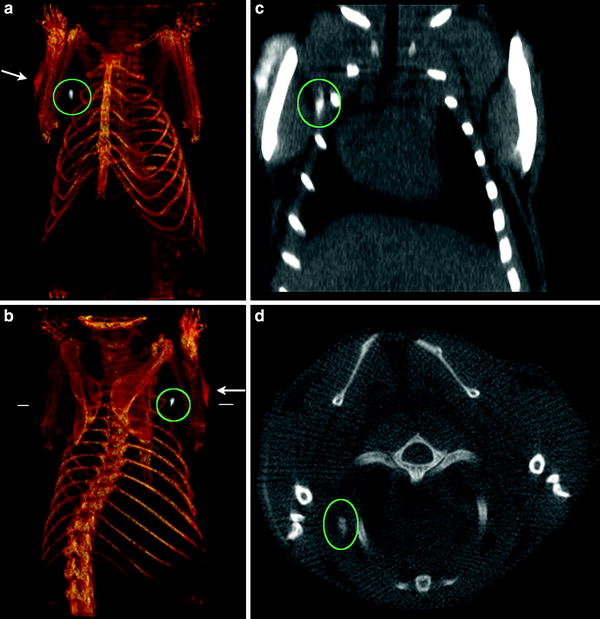
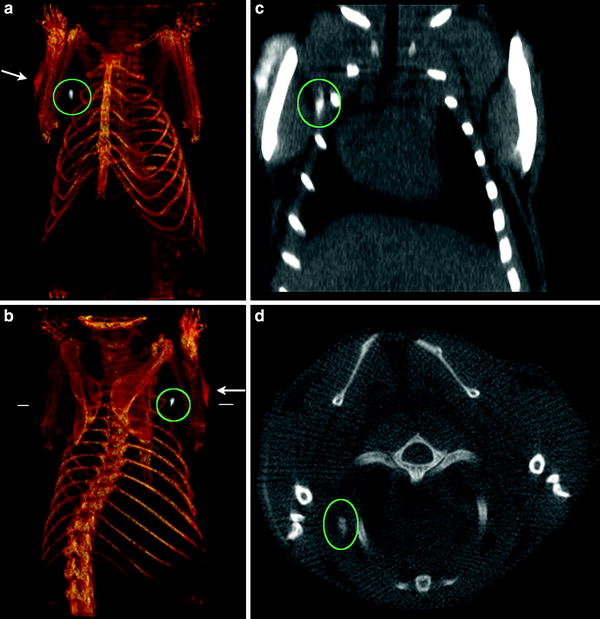
Fig. 4
CT imaging of a lymph node of a mouse with Bismuth nanoparticles. The particles have been injected subcutaneously and were transported to a regional (axillary) lymph node a, b Three-dimensional (3D) volume renderings of the CT data, the length of the reconstruction is 3.8 cm. c Coronal slice (length of the slice 2.3 cm). d Transverse slice at the level indicated by the white horizontal lines in b. The position of the lymph node (white) under the right shoulder is indicated by the green ovals, and the injection site is shown by the white arrows. Note the lack of contrast in the corresponding contralateral (left shoulder) lymph node
4 Overcoming Biological Barriers
In order to be useful as an imaging agent the Signal-to-Noise ratio (SNR) must be sufficiently high. To be detectable in living subjects, imaging probes must also have the ability to reach the intended target at a sufficient concentration within a useful time frame while at the same time be adequately cleared from the circulation and other organs (Massoud and Gambhir 2003). This makes radioactive iodine such an ideal agent as it achieves uniquely high tumor to background ratios for well-differentiated thyroid cancers (Frangioni 2009). Molecular imaging agents are subject to pharmacokinetic precepts: distribution, absorption metabolism, and elimination. Depending on its localization the target is more or less accessible (Fig. 5). To leave the vascular system, the vascular wall needs to be traversed. Imaging agents below a certain cutoff of molecular weight can leave the vascular bed easily, especially in disrupted endothelial layers as occurs within tumors. However, extravasation can be challenging, especially in the normal brain when the blood–brain barrier is intact (Begley and Brightman 2003). The interstitial space needs to be navigated to reach an interstitial target or targeted cells (Grimm and Scheinberg 2011). Additional barriers are present if the target is located in the cytoplasm of the target cells or even the nucleus, with the cell membrane and nuclear architecture (including the nuclear membrane proteins such as histones) representing additional barriers (Weissleder and Mahmood 2001; Massoud and Gambhir 2003).
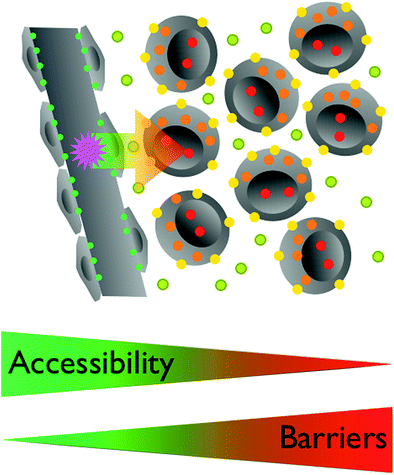
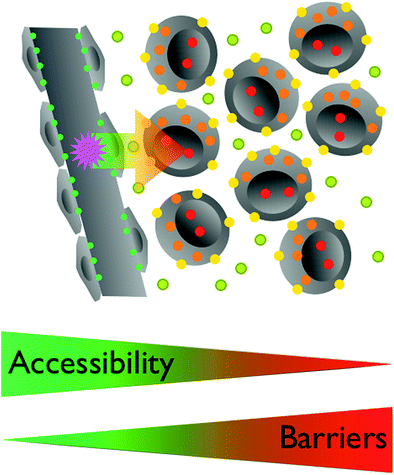
Fig. 5
The accessibility of a target by an imaging agent is related to its localization. Endothelial targets (green) are easily accessible for circulating agents. To reach interstitial targets (olive) the vascular wall has to be traversed, excluding larger particles. Targets on the cell membrane (yellow) require similar characteristics of agents targeting interstitial objectives, distribution throughout the Interstitium. For intracellular targets (orange) the cell membrane needs to be crossed, which typically requires additional modifications of the imaging agent. Nuclear targets (red) are further sequestered within the nuclear membrane. Some agents are traveling to the nucleus once in the cell, others need yet other modifications
The Enhanced Permeability and Retention (EPR) effect (certain sizes of agents tend to accumulate in tumors) can be a blessing or curse as it can either help to increase the binding or to increase nonspecific signal with increased background. The “fate” of an imaging compound in vivo depends on its properties such as molecular size, charge, solubility, avidity, affinity and (in case of nanoparticles) also loading density (Choi et al. 2010). The main process by which the body eliminates “foreign” substances is excretion. Hydrophilic materials are eliminated by the kidneys, whereas lipophilic compounds usually are metabolized by the liver, the principal organ for metabolism (Ratain et al. 1996).
5 Increasing the Signal
To visualize biological processes in vivo, it requires a very strong imaging signal per unit level of target and probe interaction due to the low amounts of target. Various signal amplification strategies have, therefore, been implemented. This is especially important if utilizing less sensitive imaging modalities or when imaging on the DNA- or mRNA-level where the number of targets per cell may be limited to a few copies. For example, there are only two copies of an individual gene, which is buried deep within the cell behinds membranes and nuclear proteins. In this respect, the strategy to image the actual product of a gene, the protein or its function (“downstream imaging”) is much more viable because of a much larger number of targets per cell, thus creating a natural amplification (Fig. 6) (Weissleder and Mahmood 2001; Massoud and Gambhir 2003). The concentration and the SNR of the imaging agent can be improved by pretargeting (Barbet et al. 1998), by avidin–biotin amplification (Rosebrough 1996; Goodwin et al. 1984), by improving kinetics (Choi 2010), or by subsequent trapping of converted ligands (Tjuvajev et al. 1996; Gambhir et al. 2000). The binding avidity to a particular target can be increased by coupling more than one ligand to the probe, creating a multivalent agent (Montet et al. 2006; Tassa et al. 2010). Another strategy is to design activatable agents (Thorek and Grimm 2012 ). Here, the imaging probe provides little or no signal in its native state and changes its physical properties after interaction with its target, which is typically an enzymatic activity (Fig. 1). Many variants of these probes have been designed for optical imaging, based on “quenching”, which describes energy transfer between neighboring fluorochromes resulting in a considerable decrease in fluorescence (Fig. 7a) (Elias et al. 2008). A classic example is the imaging of proteases such as matrix metalloproteinases (Bremer et al. 2001) or cathepsins (Fig. 3) (Grimm et al. 2005), which are all associated with tumors. Here, a peptide sequence is cleaved by the endopeptidases (Fig. 7a). Another examples are the molecular beacons, which are single stranded strands of DNA, forming a hairpin loop in themselves and thus bringing the two fluorochromes at the 3’ and 5’ end close enough together for quenching. Upon annealing to the complementary target sequence (e.g. mRNA of interest) the loop linearizes and dequenching occurs. Smart probes have been developed for MRI as well. These are based on changes of magnetic relaxation upon assembly of paramagnetic or superparamagnetic particles, resulting in a higher T1 or lower T2 signal, respectively (Fig. 7b, c) (Bogdanov et al. 2002; Perez et al. 2002). This concept has been realized as the magnetic relaxation switch with which iron oxide nanoparticles have been used to measure enzymatic activity in vitro, including proteases, restriction-endonucleases, and telomerase activity (Fig. 2 and 7d) (Grimm et al. 2004; Perez, 2002). Other activatable agents have been realized using modified Gadolinium chelates, which permitted enzyme-mediated polymerization. The mechanism of polymerization is based on the oxidation of substrates in the presence of the enzyme (such as myeloperoxidase) and hydrogen peroxide to yield free radicals, which give rise to oligomers with an increased r1 relaxivity due to a decreased tumbling time of the larger molecule (Fig. 7c) (Tannous et al. 2006; Querol et al. 2005). In an alternative scheme, enzyme-specific activation has been accomplished by “unmasking” the free gadolinium water coordination site upon galactosidase-mediated cleavage of a galactose moiety that blocked the access of bulk water to the coordination side, and thus decreased the relaxivity (Fig. 7b) (Louie et al. 2000).