(1)
where, D(z) is the radiation dose profile along the axis of rotation, N the number of data channels used in a particular axial acquisition, and T is the slice width of one data channel. The value of NT represents the total z-axis width of the active detector i.e., the beam collimation. CTDI100 is measured using a calibrated pencil ionization chamber, 10 cm long, connected to a calibrated electrometer. The integration limits (± 50 mm) correspond to the 100 mm length of the pencil ionization chamber. CTDI is expressed in Gy.
CTDI100 can be measured free-in-air or using cylindrical poly(methyl methacrylate) (PMMA) phantoms having one central and four peripheral holes for the placement of the pencil ionizing chamber (Fig. 1). Medical physicists use a 16 cm phantom to estimate the CTDI for head examinations and a 32 cm phantom to calculate the CTDI for body examinations. From CTDI100 measurements at the center and at the periphery of a PMMA phantom, CTDIW is calculated as

CTDI volume (CTDIV) is defined as
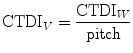
The CTDI V is independent of patient size and acquisition length. To take into account the length of the acquisition, the Dose Length Product (DLP) has been introduced, which is defined as

where SL is the scanning length. The unit of DLP is mGy·cm. The CTDI V value and/or the DLP value of CT examinations are indicated on the scanner console. Although CTDI and DLP are useful tools for patient dose management, they do not quantify the amount of patient radiation dose.
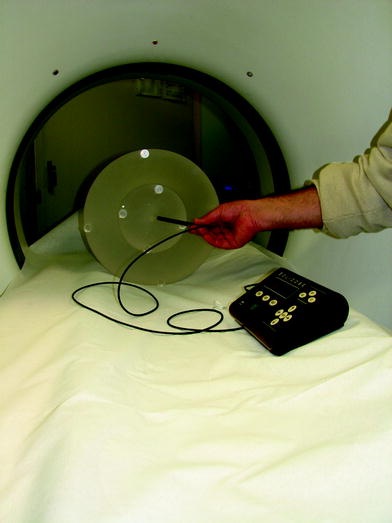

(2)
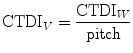
(3)

(4)
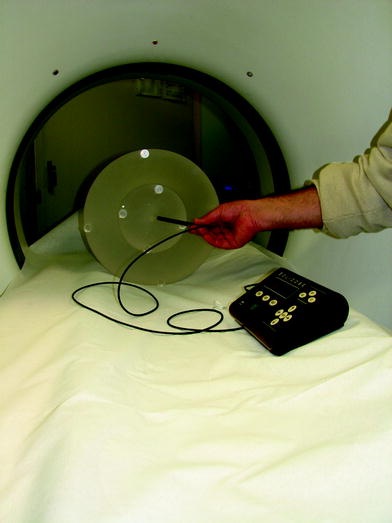
Fig. 1
Instrumentation used for CTDI determination: CTDI phantom, 10 cm long ionization chamber and electrometer
All organs and tissues of the human body are not equally sensitive to the possible biological effects of radiation such as cancer induction. To account for the different radiosensitivity of body tissues and organs, organs and tissues have been assigned weighting factor values in a recent publication by the International Commission on Radiologic Protection (ICRP) (International Commission on Radiological Protection 2007). For example, lung, colon, breast, stomach, and red bone marrow have a tissue weighting factor of 0.12, whereas the corresponding value for bladder, liver, esophagus, and thyroid is 0.04. The sum of these factors is equal to 1.0. The effective dose is calculated using
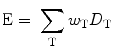
where w T is the tissue T weighting factor and D T is the absorbed dose to organ/tissue T. Effective dose is expressed in Sievert (Sv). If two or more areas have been exposed, the total body effective dose is the sum of the effective doses for each area. Therefore, the total body effective dose from a spine and a hip DXA acquisition is the sum of the effective doses from each acquisition. The effective dose is the most appropriate dose quantity to compare patient dose for different X-ray examinations, for example to compare the dose from a QCT and a DXA examination. Effective dose represent an estimate of risk from an X-ray examination.
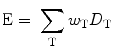
(5)
A rough estimation of patient effective dose from CT examinations is possible using the equation

where k is the normalized effective dose expressed in mSv mGy−1cm−1. Sex- and age-specific normalized effective dose values can be found in the literature (Deak et al. 2010). Patient- and scanner-specific Monte Carlo simulation methods provide accurate estimation of patient dose (Damilakis et al. 2010a, b). The output of the simulation is a series of images that depict patient radiation dose. Figure 2 shows a CT image and corresponding dose image where each pixel represents a dose value.
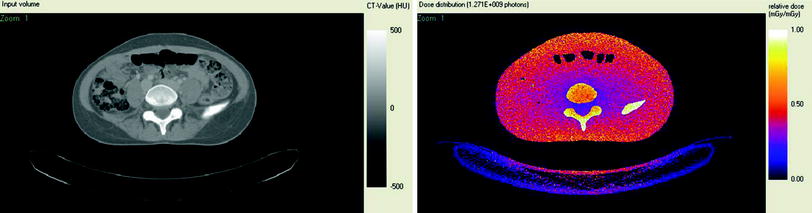

(6)
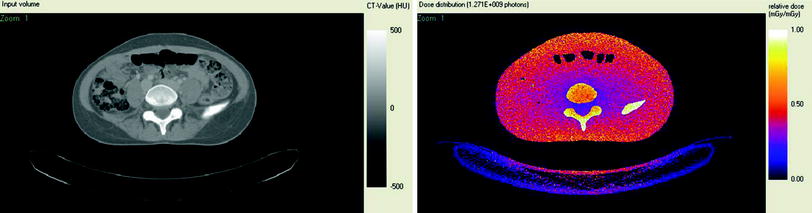
Fig. 2
A CT image (left) and the corresponding dose image generated using patient specific Monte Carlo simulation (right). Black corresponds to the lowest dose whereas white corresponds to the highest
2.2 Background Radiation Levels
Background radiation is a consistent source of low exposure natural and man-made ionizing radiation to all inhabitants of earth. Natural background radiation comes from cosmic radiation and terrestrial sources. The average effective dose from natural background radiation is 2.4 mSv per year. Man-made radiation comes from medical uses of radiation and nuclear industry. There is an increasing trend in medical radiation exposures due to the greater availability of medical radiation services. Figure 3 shows the percentage contribution of various radiation sources to the background radiation (World Nuclear Association 2011). It is evident that most radiation is from natural sources such as radon and cosmic radiation. Background radiation is sometimes used as a benchmark for judging radiation doses from medical exposures such as exposures from DXA or MDCT.
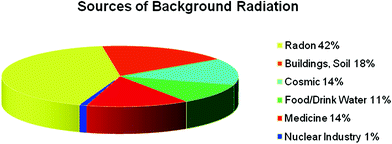
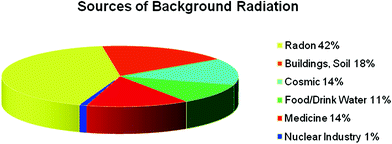
Fig. 3
The relative percentage of all sources of background radiation
2.3 Low-Level Radiation Risks
Biological effects can be categorized as deterministic or stochastic. Deterministic effects such as cataract and erythema occur when radiation dose exceeds a dose threshold. Stochastic effects occur without a threshold, their probability increases with radiation dose and consist primarily of cancer and genetic effects. The response by human beings to low-level radiation doses has been the subject of many research studies. The health effect of concern at low doses of radiation is cancer. Risk estimates are based on the linear no-threshold model. This model assumes that there is a linear relationship between radiation dose and cancer risk at all dose levels. The risks of developing radiation-induced cancer at any time subsequent to the age at exposure can be estimated using age-, sex-, and organ-specific risk data provided by the National Research Council Committee on the Biological Effects of Ionizing Radiation reports or factors provided by ICRP (International Commission on Radiological Protection 2007; National Research Council Committee on the Biological Effects of Ionizing Radiation 2006). Unborn children and children are much more radiosensitive than adults. There is evidence of cancer induction at effective doses above 5 mSv (Preston et al. 2003; Sont et al. 2001). Radiogenic risks at very low doses such as those measured during DXA examinations are uncertain. They may be zero, lower, or higher than those anticipated based on the linear no-threshold model.
2.4 The System of Radiation Protection
The radiation protection principles are justification, optimization, and dose limitation. Many X-ray examinations are unavoidable; however, it is a primary objective of medical radiation protection to keep unjustified exposures to a minimum. Justification is the process that ensures that no X-ray examination is performed unless the expected benefit to the exposed individual clearly outweighs the potential risks. Justification includes consideration of alternative examinations that use non-ionizing radiation. Exposures of pregnant patients to diagnostic X-rays as well as pediatric radiologic examinations require a higher level of justification. Screening programs for osteoporosis must be justified in terms of overall benefits and risks. Self-referral for a DXA or other bone densitometry examination should be discouraged. Optimization is the process to keep radiation exposures as low as reasonably achievable taking into account social and economic factors but still provide adequate diagnostic information. Dose limitation requires that radiation doses to the whole body or to specified organs should not exceed limits imposed by regulatory authorities. Dose limits do not apply for patient examinations, since the decision to perform an exposure is considered justified. Table 1 shows the annual limits as currently recommended by ICRP (International Commission on Radiological Protection 2007).
Table 1
The radiation dose limits recommended by the international commission on radiological protection
Type of limit | Occupational | Public (mSv/year) |
---|---|---|
Effective dose | ||
20 mSv/year | 1 | |
100 mSv in 5 years with the further provision that the effective dose should not exceed 50 mSv in a single year | ||
Absorbed dose | ||
Eye lens | 150 mSv/year | 15 |
Skin | 500 mSv/year | 50 |
Hands and feet | 500 mSv/year | – |
Embryo/fetus | 1 mGy once pregnancy has been declared | – |
2.5 Patient Radiation Doses in Bone Densitometry
2.5.1 Assessment of Osteoporotic Fractures Using Radiography, DXA and MDCT
2.5.1.1 Assessment of Osteoporotic Fractures Using Radiography
Osteoporotic fractures occur in response to low-energy trauma at the wrist, spine, and proximal femur. Conventional radiography is the standard technique for the detection of vertebral and other fractures.
Patient dose from a radiograph depends on several parameters including exposure parameters (kV, mAs), filtration, grids, the speed of the detector system, and patient body size. Over the past years, many conventional screen-film systems have been replaced by digital radiography systems. The wide dynamic range of digital detectors in combination with the capabilities of post-processing allow digital radiography systems to obtain more diagnostic information with lower patient dose. Digital radiography has several advantages over conventional screen-film radiography including ease of use. However, attention is needed to the potential increase of radiation doses due to the tendency of acquiring more images than actually needed. Another point of concern is the possibility of patient overexposure for long periods because of selection of higher exposure parameters than actually needed. Overexposure is readily visible in radiographs produced with film-screen radiography because of film blackening. With digital radiography, high exposure parameters produce excellent image quality. For this reason, newly installed digital radiography systems should be optimized to achieve the best balance between image quality and patient radiation dose. After installation, continuous patient dose monitoring is needed to ensure patient radiation protection. Table 2 shows typical effective doses for an adult patient for various radiographic examinations (Damilakis et al. 2010a, b). The actual dose for an individual patient can be two or three times higher or smaller than estimates shown in Table 2.
Table 2
Typical patient effective doses for radiographs acquired for assessment of osteoporotic fractures
Examination | Projection | Effective dose (mSv) |
---|---|---|
Thoracic spine | AP | 0.4 |
Thoracic spine | LAT | 0.3 |
Lumbar spine | AP | 0.7 |
Lumbar spine | LAT | 0.3 |
Pelvis | AP | 0.6 |
Hand | AP | 0.001 |
2.5.1.2 Assessment of Osteoporotic Fractures Using DXA
Vertebral fractures may be identified by using images acquired with DXA. Morphometric X-ray absorptiometry is a widely used method for assessment of the patient’s fracture status. The lateral projection covers the distance from T4 to L4 and the software provides information on the vertebral body heights and their ratios.
Morphometric X-ray absorptiometry is associated with a low radiation dose. Studies show that the patient effective dose ranges from 2 to 50 μSv (Vokes et al. 2006; Blake et al. 1997; Ferrar et al. 2005). The recent improvement in the image quality of DXA images in combination with low patient doses suggests that DXA is effective alternative to spine radiography for the identification of vertebral fractures.
2.5.1.3 Assessment of Osteoporotic Fractures Using MDCT
Vertebral fractures are being increasingly diagnosed fortuitously from midline sagittal reconstructions in patients having 3D multi-detector CT (MDCT) of the thorax and abdomen for other clinical reasons. In these cases, MDCT allows fracture assessment of the spine to be done without additional exposure to the patient. Bauer et al. have found that the thinnest available axial slice thickness with sagittal reconstructions of 0.6 mm performed best in fracture grading compared with conventional radiography (Bauer et al. 2006). Reconstructions based on 3 mm sections, however, also had good performance in identifying fractures. The improved spatial resolution in the longitudinal direction decreases partial volume artifacts and improves contrast depicting fine detail of the bone. When thin axial images are required, the patient dose need not be increased to obtain acceptable image noise. Thick images with good signal to noise ratios can be generated and displayed from the thinner image data.
2.5.2 Assessment of Trabecular Bone Structure Using Radiography and Texture Analysis
It is well-known that radiography is insensitive to the early and intermediate stages of osteoporosis. However, there are several features of osteoporosis that can be visualized and quantified with HR radiography and texture analysis. For this purpose, sophisticated image processing techniques such as fractal analysis or fast Fourier transforms have been used (Majumdar et al. 1999). The main limitation of these methods is that radiography records the mean absorption by all the tissues through which the X-ray beam has penetrated. Thus, cortical and trabecular bone are superimposed and the 3D bone architecture is not accurately displayed within the framework of a 2D radiograph. In vivo assessment of trabecular bone structure using radiographs is usually performed on calcaneus or distal radius images (Majumdar et al. 2000; Lespessailles et al. 2008). Lespessailles et al. have found that the combination of BMD and texture parameter values derived from calcaneus radiographs provided a better assessment of the fracture risk than obtainable only by BMDa (Majumdar et al. 2000). Patient effective dose from a calcaneus or distal radius radiograph is about 1 μSv.
2.5.3 Dual Energy X-ray Absorptiometry
DXA is based on the measurement of the transmission of photons through the body at two different X-ray energies. DXA produces dual energy photons using either rapid switching of the X-ray tube potential from 70 kVp to 140 kVp or filters developed to produce dual energy peaks by selectively removing photons at the middle of the X-ray spectrum (Damilakis et al. 2007). The detector unit discriminates between high- and low-energy photons.
Studies have focused on patient radiation dose from pencil-beam and fan-beam DXA devices (Bezakova et al. 1997; Huda and Morin 1996; Steel et al. 1998; Lewis et al. 1994; Cawte et al. 1999; Njeh et al. 1997; Steel et al. 1998; Blake et al. 2006). Patient effective dose from pencil beam DXA is about 1 μSv. With the introduction of fan-beam technology, the performance capability of DXA devices has increased considerably. However, doses to patients have also been increased. Ranges of effective doses for DXA acquisitions performed on fan-beam devices are presented in Fig. 4. The effective dose to 5-year-old children from spine or hip examinations can approach 50 μSv and 30 μSv, respectively using the default adult imaging protocol on Hologic scanners (Blake et al. 2006). However, using a pediatric protocol, pediatric doses similar to those for adult patients have been recorded. Forearm scans result in negligible dose i.e., about 1 μSv. Patient radiation doses from DXA are much lower than those from most radiologic examinations. Thus, the average effective dose from mammography is about 400 μSv, from an abdominal radiographic procedure is about 700 μSv and from head CT about 2000 μSv (Mettler et al. 2008).
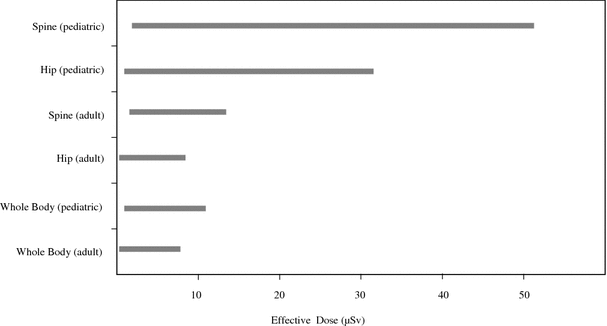
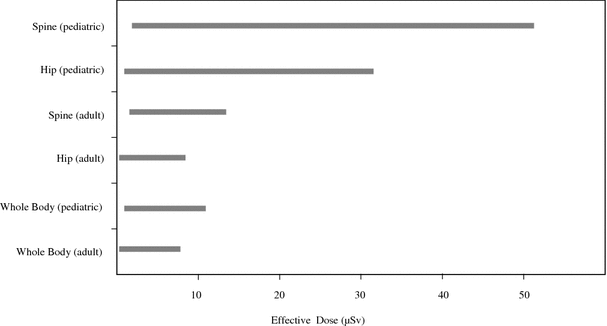
Fig. 4
Ranges of effective doses for acquisitions performed on fan-beam DXA devices
In addition to effective dose, organ radiation doses are important, especially when organs are exposed primarily, partly included or marginally excluded from the direct X-ray beam. For these organs, radiation doses from a DXA acquisition have been estimated to be from about 0.001 mGy to about 0.01 mGy (Bezakova et al. 1997; Huda and Morin 1996; Steel et al. 1998; Lewis et al. 1994; Cawte et al. 1999; Njeh et al. 1997; Blake et al. 2006). Organs receiving the greatest amount of radiation from spine DXA are bone marrow, stomach, large intestine, and ovaries. Corresponding organs for hip DXA are large intestine and testes.
The patient dose from a DXA acquisition performed on a specific DXA device depends on the acquisition mode, the body region examined, and on the patient size. Patient doses vary considerably between DXA systems of different models and manufacturers depending on several parameters such as the acquisition technology (single-sweep wide-angle vs. multi-sweep narrow-angle fan-beam systems), detector efficiency and tools developed to reduce patient dose. Even for the same examination, when different DXA systems are compared, the effective dose may vary by a factor of five or more.
With the rapid increase in the use of DXA, women of childbearing age who are unaware of their pregnancy may accidentally expose their unborn child during a DXA examination. Referring clinicians should inform radiologists before examination if their patient is pregnant. A sign that asks patients to inform staff about a possible pregnancy before the examination should be posted in the waiting area. Investigation of the reproductive status of patients of menstrual age (age range 12–50 years) is needed prior to examination. It is prudent to consider as pregnant any woman of menstrual age when a menstrual period is overdue, or missed, unless there is evidence that precludes a pregnancy (International Commission on Radiological Protection 2000). Conceptus doses from DXA performed on the mother are very low even when the unborn child is exposed primarily. Doses are lower than 5 μGy for a DXA acquisition performed using a pencil-beam device (Damilakis et al. 2002). Conceptus doses below 100 mGy should not be a reason for abortion (International Commission on Radiological Protection 2000). Therefore, termination of pregnancy due to conceptus radiation exposure during a DXA procedure is not justified. DXA examinations are rarely performed on patients known to be pregnant for the diagnosis or the differential diagnosis of pregnancy-associated osteoporosis. In these cases, the pregnant patient must understand the expected benefits and possible risks posed by the procedure. Informed consent should be obtained prior to examination.
Although radiation dose from bone densitometry techniques used in everyday clinical practice is very low, clinical justification is important for each imaging procedure and should be considered on an individual basis. A request form for a DXA examination should provide up-to-date clinical information to demonstrate the necessity for the study. In most patients, the DXA examination includes acquisitions of the hip and lumbar spine. DXA is increasingly used to estimate BMDa in children and adolescents. DXA examinations performed in children must be adjusted based on the size of the child’s body. In children, a lumbar spine and/or total body imaging usually provide enough information to answer the specific medical question. Additional skeletal sites should be imaged only when the expected benefits clearly outweigh the potential risks. The necessity for follow-up studies should be carefully considered. Multiple imaging over the years performed on the same individual for follow-up evaluation may increase radiation risks. Follow-up imaging is sometimes performed too early, when according to the known biological data; measured differences in BMDa are due solely to system variability and not to a true change in BMDa.
2.5.4 Quantitative CT and HR MDCT Imaging
QCT examinations are performed using a dedicated software package and a calibration phantom (Fig. 5) imaged simultaneously with the patient to convert the CT numbers into volumetric density (BMD; mg/cm3). In the 2D QCT protocol, three vertebral bodies (L1–L3) are measured using a single 10 mm slice through the center of each vertebra. The gantry is tilted appropriately so that the imaging plane is parallel to the vertebral endplates. The BMD measurements of the vertebrae are averaged and compared with data of a normal reference data population. Low exposure parameters (80 kVp tube potential and 120–140 mAs tube load) are used to reduce patient dose below standard CT examinations. Patient doses from 2D QCT examinations have been reported to be 60–300 μSv (Huda and Morin 1996; Kalender 1992). These dose levels, however, are above DXA dose levels.
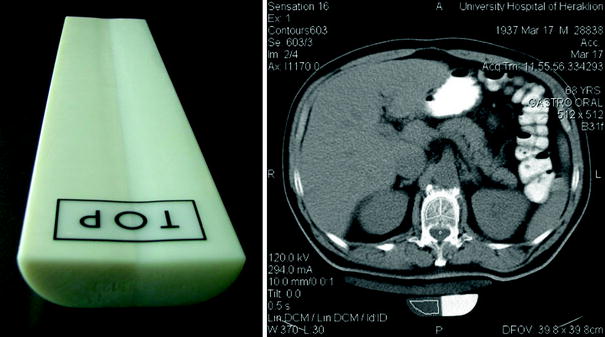
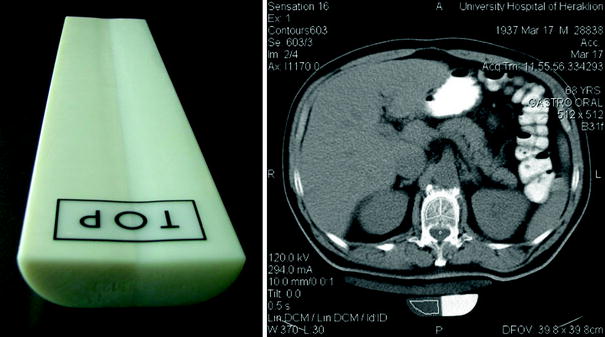
Fig. 5
The calibration phantom used in QCT (left) and CT measurement of bone mineral density and the calibration phantom positioned under the patient (right). The phantom consists of materials equivalent to soft and bone tissue
Recently, multi-detector (MD) CT protocols have been proposed to make precise measurement of BMD and bone geometry (Engelke et al. 2008, 2009a, b; Genant et al. 2008). Typical parameter settings for acquiring MDQCT data are 120 kVp, 100–150 mAs, pitch 1. An anterior-posterior scout image from the iliac crest to mid-thigh is obtained and two vertebrae (L1-L2) are usually imaged. Also, MDQCT protocols have been developed to measure BMD of the hip. Studies show that 3D MDQCT protocols of the spine and hip provide an effective dose of 1500 μSv and 2900 μSv, respectively (Engelke et al. 2008, 2009a, b; Genant et al. 2008; Khoo et al. 2009). Patient doses from MDQCT are significantly higher than doses from other methods used for estimation of BMD. Studies are needed to investigate possibilities for dose reduction while maintaining diagnostic confidence. Modern MDCT have also allowed densitometric evaluation of distal radius with good accuracy and precision. Patient effective dose from this examination is lower than 10 μSv (Engelke et al. 2009a, b). QCT at the distal radius is associated with a low radiation dose because radiosensitive organs are distant from the area being exposed primarily. However, currently peripheral QCT is most commonly performed using dedicated small peripheral QCT.
Abdominal MDCT is a frequently performed diagnostic examination that includes information on lumbar vertebrae density. Recent studies have shown that routine abdominal MDCT images can be utilized to determine lumbar spine BMD and differentiate osteoporotic from healthy individuals. Link et al. showed that there is a significant correlation in the densitometric measurements between routine spiral CT and QCT (Link et al. 2004). Quantitative CT BMD values can be derived using BMD values from routine spiral CT multiplied by a conversion factor. In a recent study, Papadakis et al. have found that QCT data derived from abdominal MDCT examinations can discriminate osteoporotic from healthy female subjects (Papadakis et al. 2009). These studies show that useful BMD information can be obtained of the lumbar spine without an additional radiation burden to the patient. However, more work is needed before routine abdominal MDCT can be considered as a method of diagnosing spinal osteoporosis.
Fast data acquisition and isotropic resolution achieved with the advent of MDCT systems has resulted in a significant increase in the use of CT in routine clinical practice over the recent years. Depending on technical parameters used, MDCT allows imaging with spatial resolution in the submillimeter range. The X-ray source-detector geometry, the field of view, the slice thickness, the reconstruction algorithms, and other factors are optimized for routine CT examinations. This limits the x-y resolution and the capability of standard MDCT to depict and quantify the 3D structure of trabecular bone. A spatial resolution better than 100 μm appears to be critical taking into account the typical dimension of trabeculae, which is 60–300 μm (Griffith and Genant 2008; Adams 2009). Although MDCT is not capable of depicting individual trabeculae, analysis of HR image provides important quantitative information regarding characteristics of the trabecular bone network (Issever et al. 2009; Krebs et al. 2009). HR MDCT is associated with patient effective doses of the order of 3000 μSv (Ito et al. 2005; Graeff et al. 2007).
During the past years, strategies have been developed for CT to deliver the lowest radiation dose to the patient necessary to obtain the information needed. Optimization of acquisition parameters (kVp, mAs, pitch, and beam collimation) may lead to a substantial decrease in patient dose from a CT examination. The use of 80 kVp for 2D QCT significantly decreases dose because of the quadratic relationship between patient dose and tube potential. An increase in tube current will proportionally increase patient dose. MDCT scanners allow automatic exposure control as the tube rotates around the patient and along z-axis. Studies have shown that the use of these tools is associated with 10–53 % reduction in tube current for adult patients and 26–43 % for pediatric patients (Greess et al. 1999, 2000, 2004 Hundt et al. 2005; Tack et al. 2003; Das et al. 2005; Gies et al. 1999; Papadakis et al. 2008). A recent study has shown that the reduction in the modulation mA may be considered as a rough approximation of the patient effective dose reduction (Papadakis et al. 2011).
The length of the acquisition should be minimal. To reconstruct the first and last slice of imaged volume of a helical CT acquisition, reconstruction algorithms require a number of extra rotations. During these additional rotations patient tissues beyond the boundaries of the area to be imaged are exposed to radiation. This feature of helical scanners is known as z overscanning. The effect of z overscanning on patient effective dose is described in detail in recent publications (Tzedakis et al. 2005, 2007). Users should maximize the distance between the boundaries of the area to be imaged and radiosensitive organs. To avoid z overscanning, axial acquisition should be selected instead of helical acquisition. Manufacturers have developed recently adaptive dose shields to reduce the effect of z overscanning (Deak et al. 2009).
2.5.5 HR Peripheral QCT
HR peripheral QCT (HR p-QCT) is a new imaging technique that assesses BMD and trabecular and cortical structural bone parameters of the radius and tibia in vivo. To improve image quality an X-ray tube with 80 μm focal spot size is employed. The spatial resolution of this technique permits quantification of bone structure characteristics since the nominal isotropic pixel dimension is 82 μm (field-of-view 12.6 cm, matrix size 1536 × 1536). Typical exposure parameters for acquiring data are 60 kVp, 900 μA and the total acquisition time is 2.8 min. Several studies have focused on this method recently to investigate bone microstructure (Burrows et al. 2010; Bacchetta et al. 2010; Rizzoli et al. 2012). HR p-QCT is a low-dose method for evaluation of bone status. The effective dose from HR p-QCT examinations is lower than 10 μSv (Engelke et al. 2008; Burrows et al. 2010).
2.5.6 Micro CT
High resolution anatomical information can be obtained using μCT and synchrotron CT usually in vitro or for small animal studies. This technology is based on principles similar to CT used in everyday clinical practice. The most important advantage of μCT systems is that acquisition of CT slices with a nominal spatial resolution to the order of down to 1 μm can be obtained. To achieve this spatial resolution, the field of measurement is much smaller than that used by clinical CT systems. As a consequence, this technology is limited to in vitro and small animal imaging because of the small bore size. A very small X-ray tube focus size is also needed to improve image quality. HR images have been used to resolve individual trabeculae and examine properties of the trabecular bone network in a manner analogous to that of histomorphometry. Furthermore, μCT has served as a gold standard to validate results of other methods (Krebs et al. 2009).
In μCT, the small tube focus size results in significantly lower X-ray tube output power. This in turn leads to prolonged acquisition times. A moderate to high radiation dose is associated with each μCT. Repeated imaging may damage organs and tissues and may have effects on the skeletal growth of living animals. Examining doses and radiation effects in different species provides information about the number of examinations each animal can undergo during its experimental lifetime. Organ radiation doses range from several mGy to a few hundreds mGy, depending upon the device model, imaging geometry, imaging protocol and method of dose estimation (Klinck et al. 2008; Brouwers et al. 2007; Obenaus and Smith 2004). Boone et al. provided normalized data for dose estimation over a range of mouse imaging geometries (Boone et al. 2004).
2.6 Occupational Radiation Doses in Bone Densitometry
During a DXA examination the patient becomes a source of scattered radiation when the X-ray beam passes through body. Assuming a workload of 20 patients per day, the annual dose at 1 m from the central axis of the examination table ranges from about 100 μSv to 1500 μSv (Larkin et al. 2008; Sheahan et al. 2005). These dose levels are much lower than the annual dose limit for staff of 20 mSv/year (International Commission on Radiological Protection 2007). Methods used to reduce patient dose will also reduce occupational dose. Additional techniques can be used to reduce staff exposure. The most effective way for the staff to reduce occupational dose from DXA is to remain as far from the patient as possible during X-ray exposure. According to inverse square law, if distance is doubled, beam intensity will decrease by a factor of four. Manufacturers provide data about the intensity and distribution of scattered radiation around the examination table (isodose curves). The intensity and distribution of scattered radiation depend on many parameters including exposure parameters, patient size and the use of shielding. Isodose curves should be taken into account to limit the risk of radiation exposure in the workplace. Installation of a DXA device requires a room with adequate size (15–20 m2) to ensure that the location of the operator is at least 2 m from the patient. In a confined space, protective shield for the operator’s console may be required for fan-beam systems. Occasionally, wall shielding may be necessary for fan-beam devices to ensure that they operate within dose limits. Certified radiation experts should assess shielding requirements and local radiation protection requirements must be strictly followed. Parameters to be taken into account in the design of protective shielding include the model of DXA device, maximum workload, the distance of the wall from the patient, the material of the walls, and the occupancy of the adjoining areas.
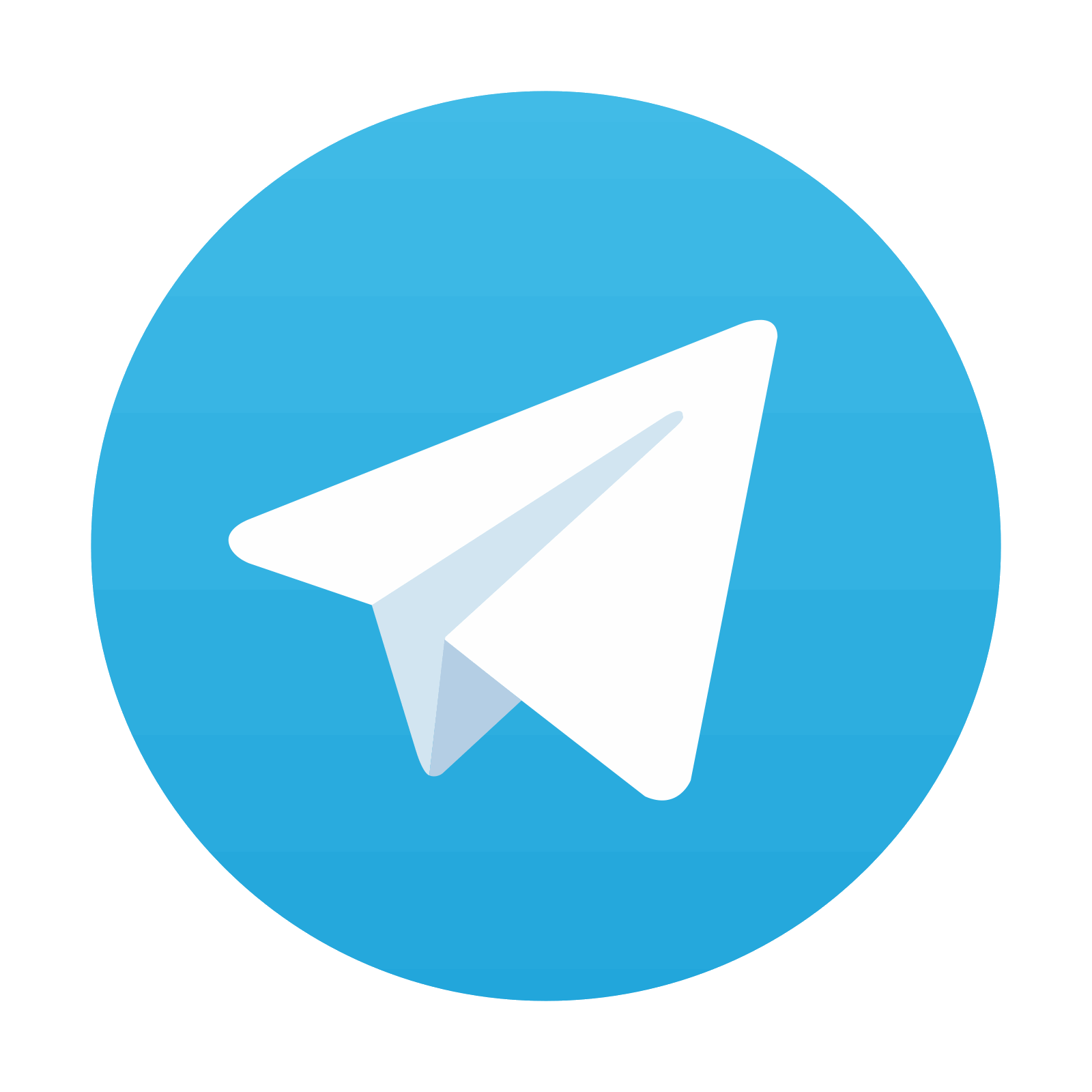
Stay updated, free articles. Join our Telegram channel
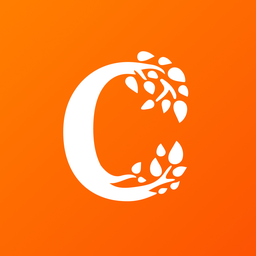
Full access? Get Clinical Tree
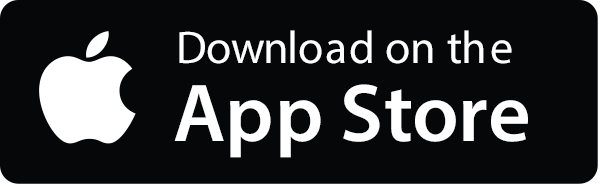
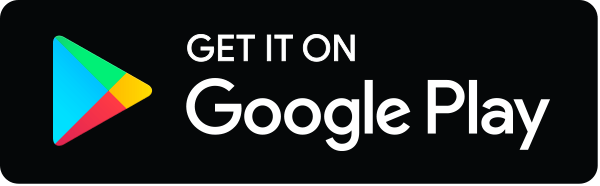