radiosensitivity of a complex biologic system is determined by a number of variables. Some of these are inherent to the type of cells exposed while others relate to the cell’s current biochemical, mitotic, and oxygen tension status as well as many other variables at the time of irradiation. Damage observed at the molecular or cellular level may or may not result in clinically detectable adverse effects. Furthermore, although some responses to radiation exposure appear instantaneously or within minutes to hours, others take weeks, years, or even decades to appear.
electrons in motion, causing additional excitation and ionization along the path of the initial energetic electron. For example, a single 30 keV electron, set in motion following the photoelectric absorption of a single x-ray or γ-ray photon, can result in the production of over 1,000 low-energy secondary electrons (referred to as delta rays), each of which may cause additional excitation or ionization events in the tissue (Goodhead, 1994). This chain of ionizations ultimately gives rise to subexcitation electrons (i.e., electrons with kinetic energies less than the first excitation potential of liquid water, 7.4 eV) that become thermalized as they transfer their remaining kinetic energy by vibrational, rotational, and collisional energy exchanges with the water molecules. Observable effects such as chromosome breakage, cell death, oncogenic transformation, and acute radiation sickness, all have their origin in radiationinduced chemical changes in important biomolecules.




complexity of clustered DNA lesions) compared with low-LET radiation (e.g., x- and γ-rays). However, beyond approximately 100 keV/µm in tissue, the RBE decreases with increasing LET, because of the overkill effect. Overkill (or wasted dose) refers to the deposition of radiation energy in excess of that necessary to produce the maximal biologic effect. The RBE ranges from less than 1 to more than 20. For a particular type of radiation, the RBE depends on the biologic endpoint being studied. For example, chromosome aberrations, cataract formation, or acute lethality of test animals may be used as endpoints. Compared to high-energy γ-rays, the increased effectiveness of diagnostic x-rays in producing DNA damage is suggested not only by the differences in their microdosimetric energy deposition patterns but has also been demonstrated experimentally with an RBE of about 1.5 to 3. However, these differences do not necessarily imply (nor have epidemiological studies been able to confirm) an associated increase in cancer risk. The RBE also depends on the total dose, dose rate, fractionation, and cell type. Despite these limitations, the RBE is a useful radiobiologic tool that helps to characterize the potential damage from various types and energies of ionizing radiation. The RBE is an essential element in establishing the radiation weighting factors (wR) discussed in Chapter 3.
destruction of the cell by radiation but rather a radiation-induced loss of mitotic capacity (i.e., reproductive death). There is considerable evidence that damage to DNA (Fig. 20-4) is the primary cause of radiation-induced cell death.
the phosphate can rejoin, provided there is no opportunity for the broken portion of the strands to separate. While the rejoining is not typically immediate, because the broken ends require the action of a series of enzymes (endonuclease, polymerase, ligase) to rejoin, the rejoining is fast and the repair typically occurs with high fidelity. The presence of oxygen potentiates the damage by causing peroxidation of a base, which then undergoes radical transfer to the sugar, causing damage that prevents rejoining.
and germ cells and, if not repaired before DNA synthesis, may be transmitted during mitosis and meiosis. Chromosomal damage that occurs before DNA replication is referred to as chromosome aberrations, whereas that occurring after DNA synthesis is called chromatid aberrations. Unlike chromosomal aberrations, in chromatid aberrations, only one of the daughter cells will be affected if only one of the chromatids of a pair is damaged.
arrest cell cycle progression) to allow for repair of damaged DNA or incompletely replicated chromosomes. In the case of potentially catastrophic DNA damage, the cell may initiate any of several cell death pathways (discussed below), effectively eliminating the damaged genetic material. The checkpoint and cell death responses (often known collectively as the DNA damage response [DDR]) utilize many of the same sensor molecules or complexes involved in DNA damage recognition and signal transduction. Many types of DNA repair mechanisms exist, including direct repair of a damaged nucleotide, base excision repair (BER), nucleotide excision repair (NER), SSB and DSB repair, and mismatch repair, each requiring its own set of enzymes (Fig. 20-6A). The repair of DNA damage depends on several factors, including the stage of the cell cycle and the type and location of the lesion.
400 mGy for chronic exposure can be detected with confidence limits that do not include zero. Although many chromosomal aberrations are unstable and gradually lost from circulation, this assay is generally considered the most sensitive method for estimating recent exposure (i.e., within 6 months). More persistent, stable reciprocal translocations can be measured using fluorescence in situ hybridization (FISH). In this method, chromosomes are labeled with chromosome-specific fluorescent DNA probes, allowing translocations to be identified using fluorescent microscopy (Fig. 20-9E). Reciprocal translocations are believed to persist for a considerable period after the exposure, and this approach has been used as one of the methods to estimate the doses to survivors of the atomic bombs detonated in Hiroshima and Nagasaki decades ago.
in the cell cycle at the time of exposure) as well as a number of physical factors related to the radiation exposure (e.g., dose, dose rate, LET), a number of responses are possible such as delayed cell division, apoptosis, reproductive failure, genomic instability (delay expression of radiation damage), DNA mutations including phenotypic (including potentially oncogenic) transformations, bystander effects (damage to neighboring unirradiated cells), and adaptive responses (irradiated cells become more radioresistant). Many of these effects are discussed in more detail below. While a wide variety of the biologic responses to radiation have been identified, the study of radiation-induced reproductive failure (also referred to as clonogenic cell death or loss of reproductive integrity) is particularly useful in assessing the relative biologic impact of various types of radiation and exposure conditions. The use of reproductive integrity as a biologic effects marker is somewhat limited, however, in that it is applicable only to proliferating cell systems (e.g., stem cells). For differentiated cells that no longer have the capacity for cell division (e.g., muscle and nerve cells), cell death is often defined as loss of specific metabolic functions or functional capacity. One must also keep in mind that, with many of the assays described below, sensitivity to detect changes may be limited at low radiation doses and dose rates, so data obtained at higher doses are often back-extrapolated, using various mathematical models discussed below, to low doses and dose rates.
Sublethal damage is a concept based on experiments that show that when the radiation dose is split into two or more fractions, with sufficient time between fractions, the cell survival increases after low-LET radiation. The presence of the shoulder in a cell survival curve is taken to indicate that more than one ionizing event (“hit”), on average, is required to kill a cell and the reappearance of the shoulder when a large dose is delivered in fractions indicates that the cells are capable of repairing sublethal damage between fractions.
![]() ▪ FIGURE 20-10 Typical cell survival curve illustrating the portions of the curve used to derive the extrapolation number (n), the quasithreshold dose (Dq), and the D0 dose. |
The LQ (or alpha-beta model, as many call it) is more commonly used than the previously described n–D0 model, for several reasons: the LQ model is mechanistically based, it is more useful in radiotherapy for explaining fractionation effect differences between late responding normal tissues and early responding tissues or tumors, and the LQ model seems to fit most experimental data on human cell lines. The dose at which cell killing is equal from the linear (αD) and quadratic (βD2) contributions is referred to as the α/β ratio. The α/β ratio is a measure of the curvature of the cell survival curve and, thus, a measure of the sensitivity of different cell types to fractionation of radiation dose, Figure 20-11. For example, late responding normal tissues such as spinal cord or lung that have smaller α/β ratios of 3 or 4 are preferentially “spared” by fractionation compared to tumors and early responding normal tissues (gut, skin, bone marrow) where the α/β ratio is larger (8 to 12), indicating less ability to repair (i.e., more alpha component and less effect of fractionating the dose).
bodies composed of cytoplasm and tightly packed organelles that are eliminated by phagocytosis. Hallmarks of apoptosis include the sequential activation of caspases (cysteine-dependent aspartate-directed proteases) from pro-caspases; interactions of pro- and anti-apoptotic members of the bcl-2 family of proteins, many working at the level of the mitochondria; cleavage of multiple proteins; and, ultimately, cleavage of DNA between nucleosomes to form characteristic fragments consisting of multiples of the amount of DNA in a nucleosome. Extrinsic apoptosis is initiated at the cell surface with activation of death receptors such as CD95 (Fas) receptor, dimerization and activation of the initiator, or upstream caspase, caspase-8, which, in turn, activates the downstream caspase-3, which activates endonucleases and other proteases
to cleave DNA and many other cellular proteins. Intrinsic apoptosis is generally started at mitochondria where interactions of pro-apoptotic proteins, such as Bax and Bak, with anti-apoptotic Bcl-2 and Bcl-XL results in the release of cytochrome c from the mitochondria, formation of apoptosomes, activation of caspase-9, activation of caspase-3, and the cleavage of other cellular proteins and DNA. Radiation can induce apoptosis through DNA damage initiating the formation of pro-apoptotic proteins such as Noxa and Puma, which activate intrinsic apoptosis or upregulation of death receptors to begin extrinsic apoptosis pathways. This is an over-simplistic description of the processes, as there can be much cross-talk among pathways and regulation by other proteins, for example, p53 or XIAP (sex-linked inhibitor of apoptosis) at various steps.
fractions of 2 Gy with sufficient time between fractions for repair of sublethal damage. For low-LET radiation, the decreasing slope of the survival curve with decreasing dose rate (see Fig. 20-14) and the reoccurrence of the shoulder with fractionation (see Fig. 20-16) are clear evidence of repair.
extremely radiosensitive compared with other cells in the body. On the other end of the spectrum, the fixed postmitotic neurons found in the central nervous system (CNS) are relatively radioresistant (Fig. 20-18). This classification scheme was refined in 1968 by Rubin and Casarett, who defined five cell types according to characteristics that affect their radiosensitivity (Table 20-1).
![]() ▪ FIGURE 20-17 Cell survival curves demonstrating the effect of oxygen during high (blue) and low (green) oxygen tension on the OER for high- and low-LET irradiation. |
phase because when it is prevented by genetic alterations or drugs such as caffeine, cells are sensitized to radiation. Also important for radiation sensitivity is the ability to arrest in the G1 phase, which is highly dependent on cells having a functional p53 pathway (see bottom of Fig. 20-19). The tumor suppressor gene TP53 (so named because it encodes a phosphorylated protein with a molecular weight of 53 kDa) operates predominantly at the G1/S checkpoint. The p53 protein (discussed again in relation to radiation-induced carcinogenesis later in the chapter) induces cellcycle arrest through the up-regulation of cyclin-dependent kinase inhibitors and thus allows for repair of DNA damage. The CIP/KIP (CDK interacting protein/Kinase inhibitory protein) family is one of two families (CIP/KIP and INK4) of mammalian cyclin-dependent kinase (CDK) inhibitors (CKIs) involved in regulating the cell cycle. The CIP/KIP family members also have a number of CDK-independent roles involving regulation of transcription, apoptosis, and the control of the cell’s cytoskeleton. Thus the p53 protein activation of cyclin-dependent kinases can activate DNA repair mechanisms or, in the case of severe DNA damage, induce cell death via apoptosis.
TABLE 20-1 CLASSIFICATION OF CELLULAR RADIOSENSITIVITY | ||||||||||||||||||||||||||||||||
---|---|---|---|---|---|---|---|---|---|---|---|---|---|---|---|---|---|---|---|---|---|---|---|---|---|---|---|---|---|---|---|---|
|
dose and dose rate as well as among lymphocytes from different individuals and with other variables. Many other endpoints for adaptive response have been studied such as cell lethality, mutations, and defects in embryonic development for which the evidence for an adaptive response was highly variable. While many theories have been advanced to explain this phenomenon, there is still insufficient evidence to use these results to modify the dose-response relationship for human exposure to radiation.
factors to predict the biological consequences of radiation exposure in humans. On the other hand, the nonlinear nature of these and other multicellular and tissue-level responses raises serious questions regarding the current paradigm of linear extrapolation of risk based on the individual cell and the target. An active area of current research focused on addressing these complex responses to radiation interactions is a multidimensional, systems-level approach that includes the integration of radiation epidemiology with radiobiological investigations.
group of soluble short-acting proteins, glycoproteins, and peptides produced by various immune and vascular cells that activate specific receptors and modulate the functions of many cells and tissues. Some cytokines may be membrane-bound or associated with ECM. Cytokines released by the vascular endothelium of irradiated tissues are implicated in the acute phase response to ionizing radiation and other inflammatory stimuli. Examples of radiation-induced cytokines include tumor necrosis factor (TNF3)-α, Interleukin (IL)-1, transforming growth factor (TGF)-β, and stem cell factor. Although many of these cytokines and growth factors, when induced by radiation, increase tissue damage, for example, the role of TGF-β in pneumonitis
is well documented, some growth factors can be radioprotective in some tissues, e.g., basic fibroblast growth factor protects microvasculature and IL-1 is a radioprotector of hematopoietic cells (Hall and Giaccia, 2018).
TABLE 20-2 RUBIN AND CASARETT CLASSIFICATION OF RELATIVE ORGAN AND TISSUE RADIOSENSITIVITY AND PRIMARY MECHANISM FOR RADIATION-INDUCED PARENCHYMAL HYPOPLASIA | ||||||||||||||||||||||||
---|---|---|---|---|---|---|---|---|---|---|---|---|---|---|---|---|---|---|---|---|---|---|---|---|
|
seen. At higher doses, radiation can interfere with normal maturation, reproduction, and repopulation of germinative epidermal cell populations. At very high doses, the mitotic activity in the germinal cells of the sebaceous glands, hair follicles, basal cell layer, and intimal cells of the microvasculature can be compromised.
|
duration of temporary sterility is dose dependent, with recovery beginning at 1 and as long as 3.5 years after doses of 1 and 2 Gy, respectively. However, following exposure (and provided the dose is not excessive), there will be a window of fertility before the onset of sterility, as long as mature sperm are available. Chronic exposures of 20 to 50 mGy/wk can result in permanent sterility when the total dose exceeds 2.5 to 3 Gy. The reduced threshold for effect following chronic versus acute exposure is unusual (i.e., an inverse fractionation effect) and is believed to be due to stem cells progressing into radiosensitive stages (Lushbaugh and Ricks, 1972). Reduced fertility due to decreased sperm count (oligospermia) and motility (asthenozoospermia) can occur 6 weeks after a dose of 150 mGy. These effects are not related to diagnostic examinations, because acute gonadal doses exceeding 100 mGy are unlikely.
TABLE 20-4 PHYSICAL AND BIOLOGICAL MODIFIERS OF RADIATION-INDUCED SKIN DAMAGE | |||||||||||||||||||||
---|---|---|---|---|---|---|---|---|---|---|---|---|---|---|---|---|---|---|---|---|---|
|
provided the exposure is not so high as to destroy the relatively radioresistant small primordial follicles. The dose that will produce permanent sterility is age dependent, with higher doses (˜10 Gy) required to produce sterility prior to puberty than in premenopausal women over 40 years old (˜2 to 3 Gy).
mSv/y to the lens of the eye may need to be reevaluated. However, the proposed ICRP limit is almost a factor of 10 lower than current limits and lower than the whole-body dose limit in the United States of 50 mSv/y. Similarly, the NCRP has recommended an occupational dose limit for the lens of the eye of 50 mGy/y (NCRP, 2016), although the U.S. national regulations have not yet been revised. Adoption of ICRP or NCRP recommendations by regulatory bodies would present new challenges for radiation protection in health care settings, especially for those involved in performing fluoroscopically guided interventional procedures. In any case, the use of eye protection in the form of leaded glasses and/or ceiling mounted lead acrylic shielding is imperative for workers whose careers will involve long-term exposure to scattered radiation.
approximately 3 weeks after the poisoning from the complications of profound pancytopenia that is characteristic of severe hematopoietic damage.
TABLE 20-5 THRESHOLD DOSES IN TISSUES AND ORGANS IN ADULTS EXPOSED TO ACUTE, FRACTIONATED OR PROTRACTED, AND CHRONIC RADIATION EXPOSUREa | ||||||||||||||||||||||||||||||||||||||||||||||||||||||||||||||||||||||||||||||||||||||||||||||||||||||||||||||||||||||||||||||||||||||||||||||||||||||||||||||||||||||||||||||||||||||||||||||||||||||||||||||||||||||||
---|---|---|---|---|---|---|---|---|---|---|---|---|---|---|---|---|---|---|---|---|---|---|---|---|---|---|---|---|---|---|---|---|---|---|---|---|---|---|---|---|---|---|---|---|---|---|---|---|---|---|---|---|---|---|---|---|---|---|---|---|---|---|---|---|---|---|---|---|---|---|---|---|---|---|---|---|---|---|---|---|---|---|---|---|---|---|---|---|---|---|---|---|---|---|---|---|---|---|---|---|---|---|---|---|---|---|---|---|---|---|---|---|---|---|---|---|---|---|---|---|---|---|---|---|---|---|---|---|---|---|---|---|---|---|---|---|---|---|---|---|---|---|---|---|---|---|---|---|---|---|---|---|---|---|---|---|---|---|---|---|---|---|---|---|---|---|---|---|---|---|---|---|---|---|---|---|---|---|---|---|---|---|---|---|---|---|---|---|---|---|---|---|---|---|---|---|---|---|---|---|---|---|---|---|---|---|---|---|---|---|---|---|---|---|---|---|
|
for approximately 2 to 4 weeks or in some cases even longer. This stage is the most difficult to manage from a therapeutic standpoint, because of the overlying immunoincompetence that results from damage to the hematopoietic system. Therefore, treatment during the first 6 to 8 weeks after the exposure is essential to optimize the chances for recovery. If the patient survives the manifest illness stage, recovery is likely; however, the patient will be at higher risk for cancer and, to a much lesser extent, his or her future progeny may have an increased risk of genetic abnormalities.
TABLE 20-6 CLINICAL FINDINGS DURING PRODROMAL PHASE OF ARS | ||||||||||||||||||||||||||||||||||||||||||||||||||||||||||||||||||||||||||||||||||||||||||||||||||||||||||||||||||
---|---|---|---|---|---|---|---|---|---|---|---|---|---|---|---|---|---|---|---|---|---|---|---|---|---|---|---|---|---|---|---|---|---|---|---|---|---|---|---|---|---|---|---|---|---|---|---|---|---|---|---|---|---|---|---|---|---|---|---|---|---|---|---|---|---|---|---|---|---|---|---|---|---|---|---|---|---|---|---|---|---|---|---|---|---|---|---|---|---|---|---|---|---|---|---|---|---|---|---|---|---|---|---|---|---|---|---|---|---|---|---|---|---|---|
|
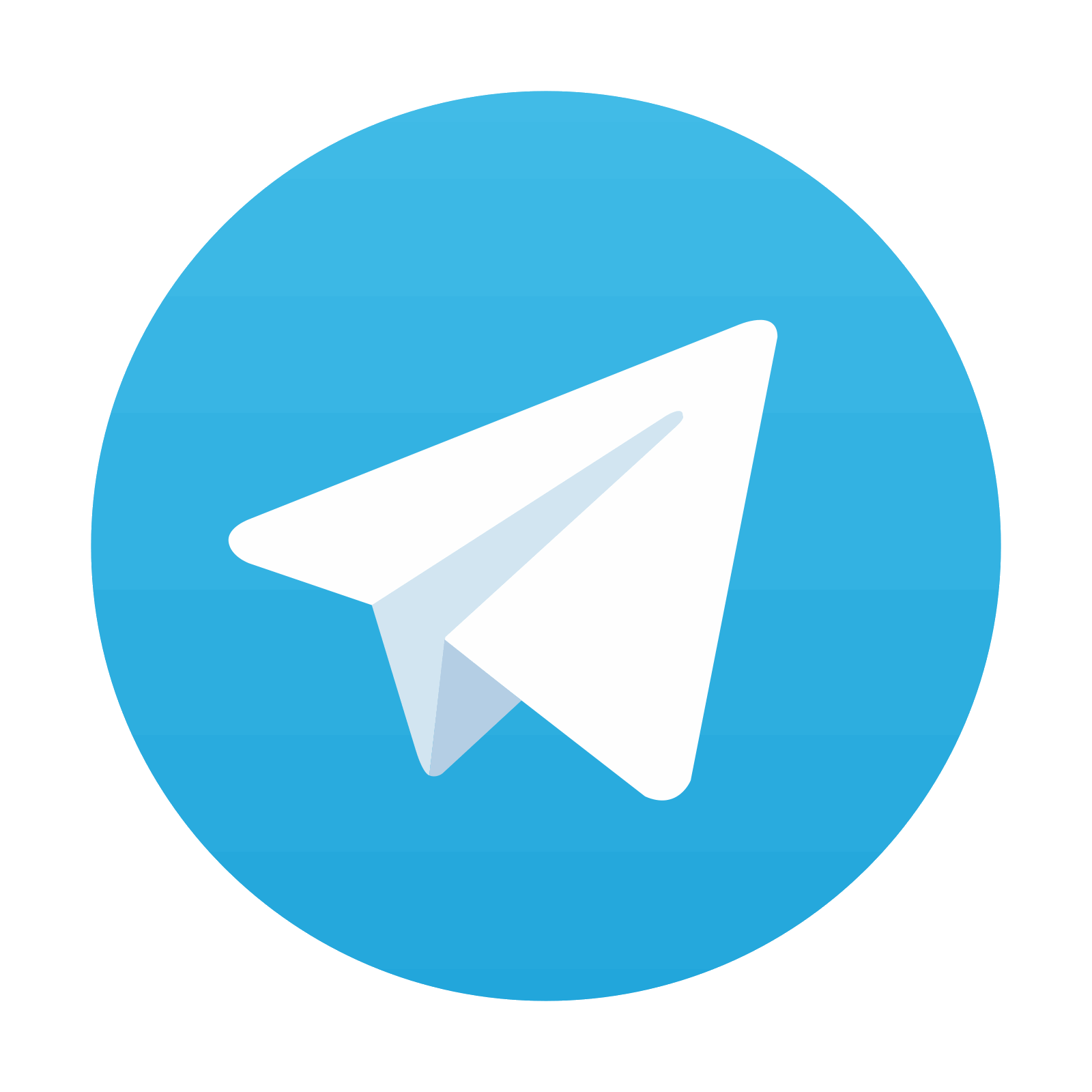
Stay updated, free articles. Join our Telegram channel
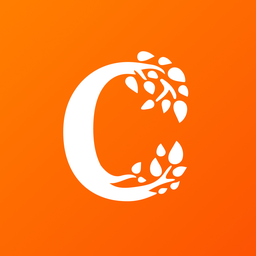
Full access? Get Clinical Tree
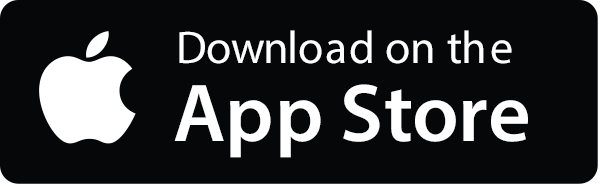
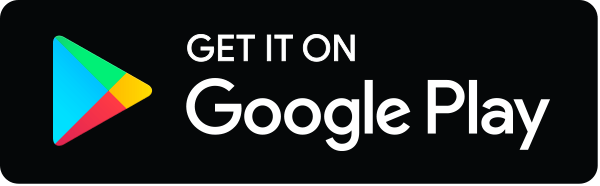