chapter 7 Radiation Detectors
When radiations from a radioactive material pass through matter, they interact with atoms and molecules and transfer energy to them. The transfer of energy has two effects: ionization and excitation. Ionization occurs when the energy transferred is sufficient to cause an orbital electron to be stripped away from its parent atom or molecule, thus creating an ion pair (a negatively charged electron and a positively charged atom or molecule). Excitation occurs when electrons are perturbed from their normal arrangement in an atom or molecule, thus creating an atom or molecule in an excited state. Both of these processes are involved in the detection of radiation events; however, ionization is the primary event, and hence the term ionizing radiation is used frequently when referring to the emissions from radioactive material. Radiation interactions were discussed in detail in Chapter 6. In this chapter, we describe the basic principles of radiation detectors used in nuclear medicine.
a Gas-Filled Detectors
1 Basic Principles
Most gas-filled detectors belong to a class of detectors called ionization detectors. These detectors respond to radiation by means of ionization-induced electrical currents. The basic principles are illustrated in Figure 7-1. A volume of gas is contained between two electrodes having a voltage difference (and thus an electric field) between them. The negative electrode is called the cathode, the positive electrode the anode. The electrodes are shown as parallel plates, but they may be a pair of wires, concentric cylinders, and so forth. Under normal circumstances, the gas is an insulator and no electrical current flows between the electrodes. However, radiation passing through the gas causes ionization, both direct ionization from the incident radiation and secondary ionization from δ rays (see Chapter 6, Section A.1). The electrons produced by ionization are attracted to the positive electrode and the ionized atoms to the negative electrode, causing a momentary flow of a small amount of electrical current.
Gas-filled detectors include ionization chambers, proportional counters, and Geiger-Müller (GM ) counters. The use of these detectors in nuclear medicine is somewhat limited because their stopping power and detection efficiency for x rays and γ rays are quite low; however, they find some use for applications in which detection efficiency is not a major factor and for detection and measurement of nonpenetrating, particle-type radiations. Some of their applications are discussed in Chapters 12 and 23.
2 Ionization Chambers
For maximum efficiency of operation, the voltage between the electrodes must be sufficient to ensure complete collection of ions and electrons produced by radiation within the chamber. If the voltage is too low, some of the ions and electrons simply recombine with one another without contributing to electrical current flow. Figure 7-2 shows the effect of voltage difference between the electrodes on the electrical current recorded by an ionization chamber per ionizing radiation event detected. Recombination occurs at low voltages (recombination region of the curve). As the voltage increases there is less recombination and the response (electrical current) increases. When the voltage becomes sufficient to cause complete collection of all of the charges produced, the curve enters a plateau called the saturation region. The voltage at which the saturation region begins is called the saturation voltage (Vs). Typically, Vs ≈ 50-300 V, depending on the design of the chamber. Ionization chambers are operated at voltages in the saturation region. This ensures a maximum response to radiation and also that the response will be relatively insensitive to instabilities in the voltage applied to the electrodes.
The amount of electrical charge released in an ionization chamber by a single ionizing radiation event is very small. For example, the energy expended in producing a single ionization event in air is approximately 34 eV.* Thus a 1-MeV β particle, for example, causes approximately (106/34) ≈ 3 × 104 ionizations in air and releases a total amount of electrical charge of only approximately 3 × 10−15 coulombs.
Because of the small amount of electrical charge or current involved, ionization chambers generally are not used to record or count individual radiation events. Instead, the total amount of current passing through the chamber caused by a beam of radiation is measured. Alternatively, the electrical charge released in the chamber by the radiation beam may be collected and measured.
Small amounts of electrical current are measured using sensitive current-measuring devices called electrometers. Two devices consisting of ionization chambers and electrometers in nuclear medicine are survey meters and dose calibrators. A typical ionization chamber survey meter is shown in Figure 7-3. The survey meter is battery operated and portable. The ionization chamber consists of an outer cylindrical electrode (metal or graphite-coated plastic) with a wire electrode running down its center. There is often a protective cap on the end of the chamber for most measurements; however, it is removed for measurement of nonpenetrating radiations such as α particles, β particles, and low-energy (10 keV) photons.
Survey meters are used to monitor radiation levels for radiation protection purposes (see Chapter 23, Section E). Ionization current is displayed on a front-panel meter. Many older units are calibrated to read traditional units of exposure rate in roentgens per hour (R/hr) or mR/hr. Newer units are calibrated to read Systeme International units of air kerma in grays per hour (Gy/hr), mGy/hr, and so forth, or have a switch-selectable option for choosing between the two systems of units. The definitions and relationships between these units are discussed in Chapter 23. A typical survey meter can measure exposure rates down to approximately 1 mR/hr or air kerma rates down to approximately 10 µGy/hr.
Dose calibrators are used to assay activity levels in syringes, vials, and so forth containing materials that are to be administered to patients. Unlike other types of ionization chambers discussed in this section, dose calibrators employ sealed and pressurized chambers filled with argon gas. This eliminates the effect of changing barometric pressure on output readings. Dose calibrators typically are calibrated to read directly in units of activity (becquerels or curies), with switches to set the display for different radionuclides. Dose calibrators are discussed in detail in Chapter 12, Section D.1.
A device that records total charge collected over time is the pocket dosimeter. The basic principles are illustrated in Figure 7-4. The ionization chamber electrodes are a central charging electrode and the outside case of the dosimeter. They are insulated electrically from one another and form an electrical capacitor. The capacitor is first charged to a reference voltage V by connecting the charging rod to a separate charging unit. If the capacitance between the charging electrode and the case is C, the charge stored on the capacitor is Q = V × C. When the chamber is exposed to radiation, electrical charge ΔQ is collected by the electrodes, discharging the capacitor. The voltage change across the capacitor is measured and is related to the amount of electrical charge collected by the ionization chamber electrodes (ΔQ = ΔV × C).
Pocket dosimeters are used in nuclear medicine to monitor radiation levels for radiation protection purposes. A typical system is shown in Figure 7-5. The ionization chamber is contained in a small metal or plastic cylinder (~1.5 cm diameter × 10 cm long) that can be clipped to a shirt pocket or collar. Electrodes recessed into one end of the chamber are used to connect the dosimeter to a separate charger unit to charge up the capacitor to the reference voltage. Voltage on the capacitor causes a fine wire within the chamber to be deflected. The position of the wire changes as the voltage on the capacitor changes. The wire is observed through a viewing window at one end of the chamber. Its position is read against a scale that has been calibrated in terms of the total radiation recorded by the chamber, usually in units of air kerma (gray) or exposure (roentgens) (see Chapter 23, Section E). Pocket dosimeters are suitable for measuring radiation exposures down to approximately 10 mR (air kerma of 0.1 mGy) to an accuracy of approximately 20%.
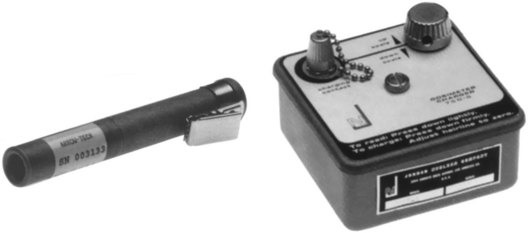
FIGURE 7-5 Pocket dosimeter with charging system.
(Courtesy Ludlum Measurements Inc., Sweetwater, Tx.)
Two additional problems with ionization chambers should be noted. The first is that for x rays and γ rays, their response changes with photon energy because photon absorption in the gas volume and in the chamber walls (i.e., detection efficiency) and relative penetration of photons through the chamber walls are both energy-dependent processes. Figure 7-6 shows a typical energy-response curve for a survey meter. A second problem is that in unsealed chambers the density of the air in the chamber, and hence its absorption efficiency, changes with atmospheric pressure (ρ ∝ P) and temperature (ρ ∝ 1/T). Most chambers are calibrated to read accurately at sea-level pressure (Pref = 1.013 N/m2 = 760 mm Hg) and average room temperature (Tref = 22°C = 295K). For other temperatures T and pressures P the chamber reading must be corrected (multiplied) by a temperature-pressure correction factor
3 Proportional Counters
In an ionization chamber, the voltage between the electrodes is sufficient only to collect those charges liberated by direct action of the ionizing radiations. However, if the voltage is increased to a sufficiently high value, the electrons liberated by radiation gain such high velocities and energies when accelerated toward the positive electrode that they cause additional ionization in collisions with other atoms in the gas. These electrons in turn can cause further ionization and so on. This cascade process is called the Townsend avalanche or the gas amplification of charge. The factor by which ionization is increased is called the gas amplification factor. This factor increases rapidly with applied voltage, as shown in Figure 7-7. The gas amplification factor may be as high as 106, depending on the chamber design and the applied voltage.
Detectors that operate in the ascending portion of the curve shown in Figure 7-7 are called proportional counters. In this region, the ionization caused by an incident radiation event is multiplied (amplified) by the gas amplification factor. The total amount of charge produced is equal to the number of ionizations caused by the primary radiation event (at 34 eV/ionization in air) multiplied by the amplification factor. Thus the total charge produced is proportional to the total amount of energy deposited in the detector by the detected radiation event.
Actually, proportional counters are not simply ionization chambers operated at high voltages but are specially constructed chambers designed to optimize the gas amplification effect, both in terms of the amount of amplification and the uniformity of this amplification within the chamber. In particular, proportional counters are filled with gases that allow easy migration of free electrons, because this is critical for the amplification effect. Common fill gases are the noble gases, with argon and xenon being the most popular.
The major advantage of proportional counters versus ionization chambers is that the size of the electrical signal produced by an individual ionizing radiation event is much larger. They are, in fact, useful for detecting and counting individual radiation events. Furthermore, because the size of an individual current pulse is proportional to the amount of energy deposited by the radiation event in the detector, proportional counters can be used for energy-sensitive counting, such as to discriminate between radiation events of different energies on the basis of electrical pulse size (see Chapter 10). They are still inefficient detectors for higher energy x rays and γ rays. Consequently, they find very limited use in nuclear medicine. Proportional counters are used mostly in research applications for measuring nonpenetrating radiations such as α particles and β particles. A practical application is discussed in Chapter 12, Section D.2.
4 Geiger-Müller Counters
A Geiger-Müller (GM) counter is a gas-filled detector designed for maximum gas amplification effect. The principles of a GM counter are shown in Figure 7-8. The center wire (anode) is maintained at a high positive voltage relative to the outer cylindrical electrode (cathode). The outer electrode may be a metal cylinder or a metallic film sprayed on the inside of a glass or plastic tube. Some GM counters have a thin radiation entrance window at one end of the tube. The cylinder of the tube is sealed and filled with a special gas mixture, typically argon plus a quenching gas (discussed later).
When ionization occurs in a GM counter, electrons are accelerated toward the center wire. Gas amplification occurs in the GM counter as in a proportional counter. In addition to ionizing gas molecules, the accelerating electrons also can cause excitation of gas molecules through collisions. These excited gas molecules quickly (~10−9 sec) return to the ground state through the emission of photons at visible or ultraviolet (UV) wavelengths. If a UV photon interacts in the gas, or at the cathode surface by photoelectric absorption (see Chapter 6, Section C.2), this releases another electron, which can trigger a further electron avalanche as it moves toward the anode (see Fig. 7-8). In this way, an avalanche ionization is propagated throughout the gas volume and along the entire length of the center wire.
As the avalanche progresses, the electrons, being relatively light, are quickly collected, but the heavy, slow-moving positive ions are not. Eventually, a “hose” of slow-moving positive charges is formed around the center wire. The avalanche then terminates because the positive ions reduce the effective electric field around the anode wire, eventually dropping it below the level required for gas multiplication.
The avalanche ionization in a GM tube releases a large and essentially constant quantity of electrical charge, regardless of voltage applied to the tube (Fig. 7-9) or the energy of the ionizing radiation event. The gas amplification factor may be as high as 1010. The large electrical signal is easily detected with electronic circuits. Thus a GM counter, like a proportional counter, can be used to detect and count individual ionizing radiation events. However, because the size of the electrical signal output is constant, regardless of the energy of the radiation detected, a GM counter cannot be used to distinguish between radiation events of different energies.
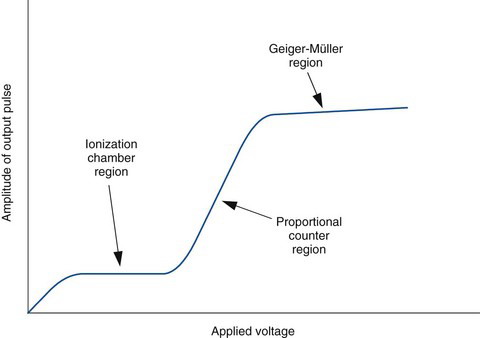
FIGURE 7-9 Voltage response curve (pulse amplitude vs. applied voltage) for a Geiger-Müller counter.
This problem is prevented by the introduction of a quenching gas into the GM counter gas mixture. Such GM counters are called self-quenched. Effective quenching gases have three properties: First, they tend to give up electrons easily. When the positive ion cloud is formed, molecules of the quenching gas neutralize other ions by donating electrons to them. The ion cloud is thus converted into ionized molecules of quenching gas. Second, when the quenching gas molecules are neutralized by electrons entering higher energy orbits, they deenergize themselves by dissociating into molecular fragments rather than by emitting UV photons. Third, the quenching gas molecules are strong absorbers of UV radiation. Thus the few UV photons that are released during neutralization of the positive ion cloud are quickly absorbed before they can set off another avalanche.
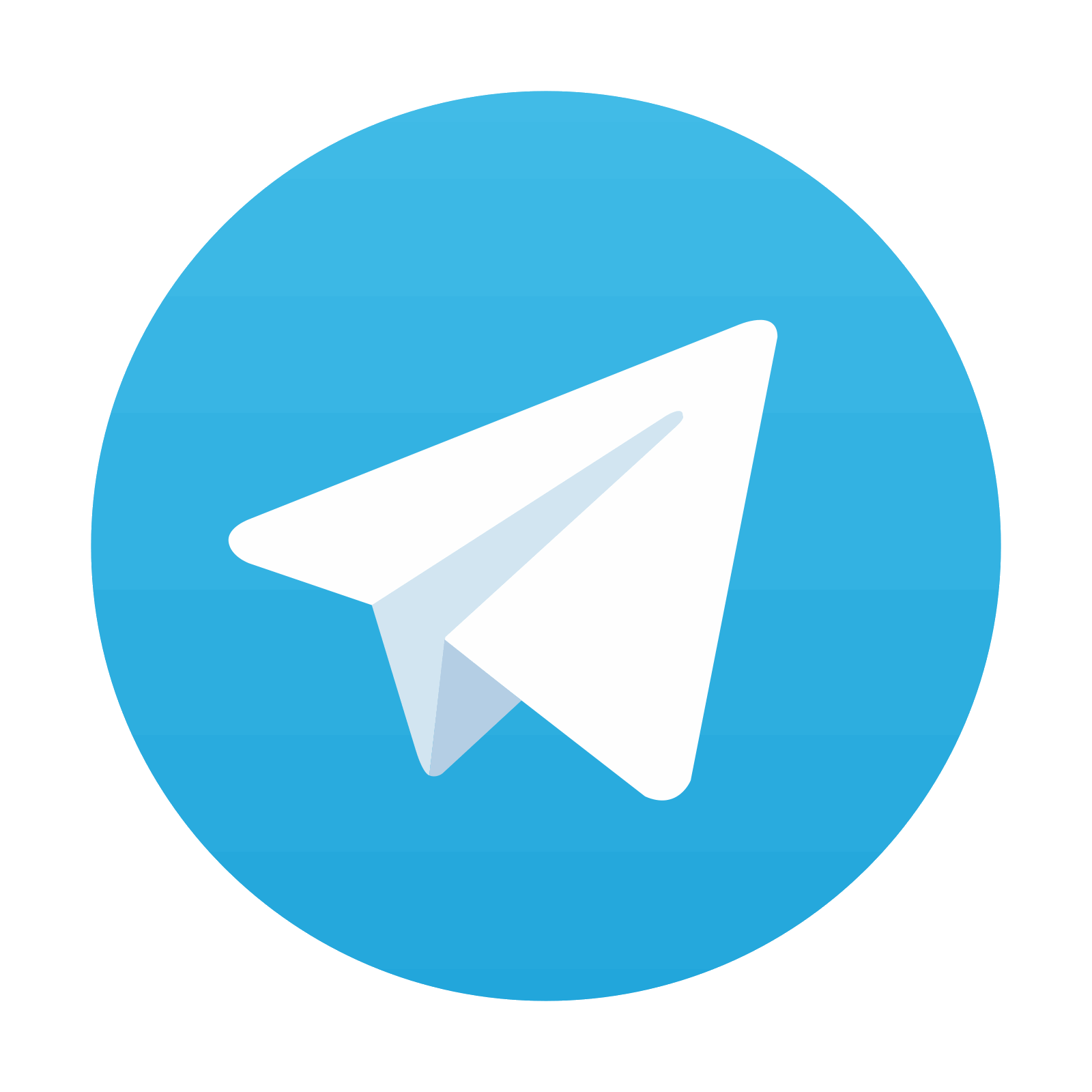
Stay updated, free articles. Join our Telegram channel
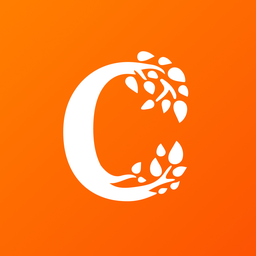
Full access? Get Clinical Tree
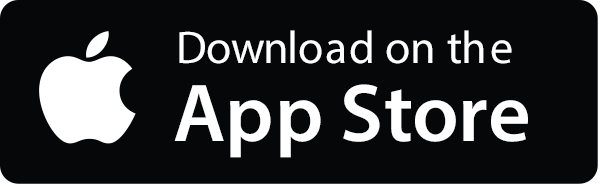
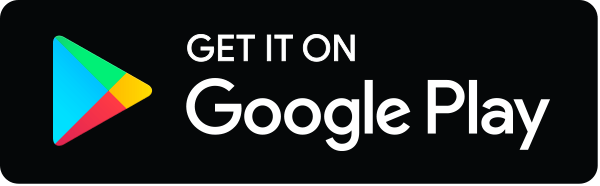