36 Radiation oncology,* or radiation therapy, is one of three principal modalities used in the treatment of cancer. The others are surgery and chemotherapy. In radiation therapy for malignancies, tumors or lesions are treated with cancericidal doses of ionizing radiation as prescribed by a radiation oncologist, a physician who specializes in the treatment of malignant disease with radiation. The goals of the treatment are to deliver a cancericidal dose of radiation precisely to the tumor, limiting as much as possible the dose of radiation received by normal, noncancerous tissues. These dual tasks make this form of treatment complex and often challenging. Input from all members of the radiation oncology team is crucial in developing the optimal treatment plan or approach for a patient. • When the surgical margin between normal tissue and cancerous tissue is minimal (<2 cm) • When the margin is positive for cancer, (i.e., when cancerous tissue is not completely removed) • When the tumor is incompletely resected because of its large size or its relationship with normal vital structures, or both Ionizing radiation was first applied for the treatment of a more in-depth lesion on January 29, 1896, when Grubbé is reported to have irradiated a woman with carcinoma of the left breast. This event occurred only 3 months after the discovery of x-rays by Röntgen (Table 36-1). Although Grubbé neither expected nor observed any dramatic results from the irradiation, the event is significant simply because it occurred. The most common cancers that occur in the United States are lung, prostate, breast, and colorectal cancer. Prostate cancer is the most common malignancy in men, and breast cancer is the most common malignancy in women. The second and third most common cancers in men and women are lung and colorectal cancer (Table 36-2). Many factors can contribute to a person’s potential for the development of a malignancy. These factors can be external exposure to chemicals, viruses, or radiation within the environment or internal factors such as hormones, genetic mutations, and disorders of the immune system. Cancer commonly is the result of exposure to a carcinogen, which is a substance or material that causes cells to undergo malignant transformation and become cancerous. Some known carcinogenic agents are listed in Table 36-3. Cigarettes and other tobacco products are the principal cause of cancers of the lung, esophagus, oral cavity and pharynx, and bladder. Cigarette smokers are 10 times more likely to develop lung cancer than nonsmokers. Occupational exposure to chemicals such as chromium, nickel, or arsenic can also cause lung cancer. A person who smokes and works with chemical carcinogens is at even greater risk for developing lung cancer than a nonsmoker. In other words, risk factors can have an additive effect, acting together to initiate or promote the development of cancer. TABLE 36-3 Carcinogenic agents and the cancers they cause Cancers may arise in any human tissue. Tumors are usually categorized under six general headings according to their tissue of origin (Table 36-4). Of cancers, 90% arise from epithelial tissue and are classified as carcinomas. Epithelial tissue lines the free internal and external surfaces of the body. Carcinomas are subdivided further into squamous cell carcinomas and adenocarcinomas based on the type of epithelium from which they arise. A squamous cell carcinoma arises from the surface (squamous) epithelium of a structure. Examples of surface epithelium include the oral cavity, pharynx, bronchus, skin, and cervix. An adenocarcinoma is a cancer that develops in glandular epithelium such as in the prostate, colon and rectum, lung, breast, or endometrium. To facilitate the exchange of patient information from one physician to another, a system of classifying tumors based on anatomic and histologic considerations was designed by the International Union Against Cancer and the American Joint Committee for Cancer (AJCC) Staging and End Results Reporting. The AJCC TNM classification (Table 36-5) describes a tumor according to the size of the primary lesion (T), the involvement of the regional lymph nodes (N), and the occurrence of metastasis (M). TABLE 36-5 Application of TNM classification system Note: This is a generalization. Variations of the staging system exist for each tumor site. As the LET increases, so does the RBE. RBE and LET values are listed in Table 36-6. TABLE 36-6 Relative biologic effectiveness and linear energy transfer values for certain forms of radiation The greater the number of interactions that occur, the greater the possibility of cell death. The preceding information leads to a categorization of tumors according to their radiosensitivity: • Gonadal germ cell tumors (seminoma of testis, dysgerminoma of ovary) • Lymphoproliferative tumors (Hodgkin and non-Hodgkin lymphomas) EXTERNAL-BEAM THERAPY AND BRACHYTHERAPY 1. Mold technique—placement of a radioactive source or sources on or in close proximity to the lesion 2. Intracavitary implant technique—placement of a radioactive source or sources in a body cavity (i.e., uterine canal and vagina) 3. Interstitial implant technique—placement of a radioactive source or sources directly into the tumor site and adjacent tissue (i.e., sarcoma in a muscle) • 120-kVp superficial x-ray unit for treating lesions on or near the surface of the patient • 250-kVp orthovoltage x-ray unit for moderately superficial tissues • 60Co (cobalt-60) gamma ray source with an average energy of 1.25-MeV; Gamma Knife • 6-MV to 35-MV linear accelerator to serve as a source of high-energy (megavoltage) electrons and x-rays The dose depositions of these units are compared in Fig. 36-1. The skin-sparing effect, a phenomenon that occurs as the energy of a beam of radiation is increased, is valuable from a therapeutic standpoint. In the superficial and orthovoltage energy range, the maximum dose occurs on the surface of the patient, and deposition of the dose decreases as the beam traverses the patient. As the energy of the beam increases into the megavoltage range, the maximum dose absorbed by the patient occurs at some point below the skin surface. The skin-sparing effect is important clinically because the skin is a radiosensitive organ. Excessive dose deposition to the skin can damage the skin, requiring treatments to be stopped and compromising treatment to the underlying tumor. The greater the energy of the beam, the more deeply the maximum dose is deposited (Fig. 36-2). The use of 60Co units has declined significantly since the 1980s, and 60Co is rarely used for conventional external-beam radiation therapy today. This decline has been basically attributed to the introduction of the more sophisticated linac, which has greater skin-sparing capabilities and more sharply defined radiation fields. The radiation beam, or field, from a 60Co unit also has large penumbra, which results in fuzzy field edges, another undesirable feature. 60Co is still used in radiation oncology as part of a special procedure called stereotactic radiosurgery. The treatment unit is called the Gamma Knife. The Gamma Knife consists of 192 to 201 60Co sources arranged in a hemispherical array with all sources converging at a single point (Fig. 36-3). The point where the beams converge forms a treatment area of 4 to 18 mm in diameter.
RADIATION ONCOLOGY
Principles of Radiation Oncology
Historical Development
Cancer
RISK FACTORS
Carcinogen
Resultant cancer
Cigarette smoking
Cancers of lung, esophagus, bladder, and oral cavity/pharynx
Arsenic, chromium, nickel, hydrocarbons
Lung cancer
Ultraviolet light
Melanoma and nonmelanomatous skin cancers
Benzene
Leukemia
Ionizing radiation
Sarcomas of bone and soft tissue, skin cancer, and leukemia
TISSUE ORIGINS OF CANCER
Classification
Description of tumor
Stage 0—T0N0M0
Occult lesion; no evidence clinically
Stage I—T1N0M0
Small lesion confined to organ of origin with no evidence of vascular and lymphatic spread or metastasis
Stage II—T2N1M0
Tumor of <5 cm invading surrounding tissue and first-station lymph nodes but no evidence of metastasis
Stage III—T3N2M0
Extensive lesion >5 cm with fixation to deeper structure and with bone and lymph invasion but no evidence of metastasis
Stage IV—T4N3M1
More extensive lesion than above or with distant metastasis (M1)
Theory
Technical Aspects
EQUIPMENT
Cobalt-60 units
RADIATION ONCOLOGY
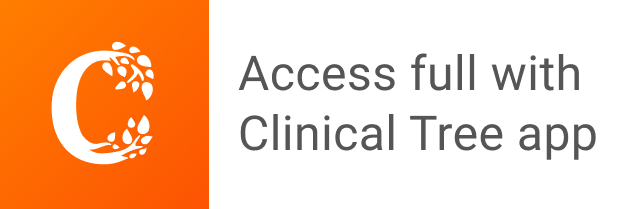