the time interval between accelerations remains constant. The cyclic nature of these events led to the name “cyclotron.” The final kinetic energy achieved by the accelerated particles depends on the type of particle (e.g., protons or deuterons), the diameter of the dees, and the strength of the static magnetic field. Finally, as the ions reach the periphery of the dees, they are removed from their circular path by a negatively
charged deflector plate (if positive ions are accelerated) or electron stripping foil (if H− ions are accelerated), emerge through the window, and strike the target. Depending on the design of the cyclotron, particle energies can range from a few million electron volts (MeV) to several hundred MeV.



imaging facilities. Production methods of clinically useful positron-emitting radionuclides are listed below.

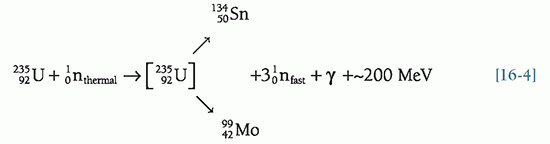
produced by the fission process (Fig. 16-4). These fission products are neutron-rich and therefore almost all of them decay by beta-minus (β−) particle emission.
reducing the reactivity (i.e., causing the neutron fluence rate and power output to decrease with time). Removing the control rods has the opposite effect. If a nuclear reactor accident results in loss of the coolant, the fuel can overheat and melt (socalled meltdown). However, because of the design characteristics of the reactor and its fuel, an atomic explosion, like those from nuclear weapons, is impossible.
![]() ▪ FIGURE 16-5 Schematic of a nuclear chain reaction. The neutrons (shown as small blackened circles) are not drawn to scale with respect to the uranium atoms. |
reactor vessel. These components, together with other highly radioactive reactor systems, are enclosed in a large steel-reinforced concrete shell (˜1 to 2 m thick), called the containment structure. In addition to serving as a moderator and coolant, the water in the reactor acts as a radiation shield, reducing the radiation levels adjacent to the reactor vessel. Specialized nuclear reactors are used to produce clinically useful radionuclides from fission products or neutron activation of stable target material.
the radionuclide present. Thus, the concentration or specific activity (measured in MBq or Ci per gram) of these “carrier-free” fission-produced radionuclides is very high. High-specific-activity, carrier-free nuclides are preferred in radiopharmaceutical preparations to increase the labeling efficiency of the preparations and minimize the mass and volume of the injected material.


a weekly supply. This supply problem is overcome by obtaining the parent Mo-99, which has a longer half-life (67 h) and continually produces Tc-99m. The Tc-99m is collected periodically in sufficient quantities for clinical operations. A system for holding the parent in such a way that the daughter can be easily separated for clinical use is called a radionuclide generator.
TABLE 16-1 COMPARISON OF RADIONUCLIDE PRODUCTION METHODS | ||||||||||||||||||||||||||||||||||||||||||||
---|---|---|---|---|---|---|---|---|---|---|---|---|---|---|---|---|---|---|---|---|---|---|---|---|---|---|---|---|---|---|---|---|---|---|---|---|---|---|---|---|---|---|---|---|
|
use of a bacteriostatic agent in the eluant, or by autoclave sterilization of the loaded column by the manufacturer.
![]() ▪ FIGURE 16-7 A. Picture of a “wet” molybdenum 99/technetium 99m generator in the process of being eluted (left). A spent generator that is no longer radioactive was used in order to minimize dose. For picture clarity, the shielding normally surrounding the generator is not shown [as illustrated in the accompanying diagram (right)]. However, correct radiation safety principles (discussed further in Chapter 21) are shown including the use of disposable gloves, finger ring and body dosimeters, and disposable plastic backed absorbent paper on the bench top to minimize the spread of any contamination. An explosion diagram depicting the generator components, and auxiliary radiation shielding is shown on the right. B. A cross-sectional diagram of the generator interior and column detail. Consult the text for additional information on the elution process. (Adapted from photo and diagrams provided courtesy of Curium US LLC, St. Louis, MO.) |
The activity of the parent
The rate of formation of the daughter, which is equal to the rate of decay of the parent (i.e., A0e-λpt)
The decay rate of the daughter
The time since the last elution
The elution efficiency (typically 80% to 90%)
Therefore, at equilibrium, the Tc-99m activity will be only approximately 97% (1.1 × 0.88) that of the parent (Mo-99) activity.
the parent. The strontium-82/rubidium-82 (Sr-82/Rb-82) generator, with parent and daughter half-lives of 25.5 d and 75 s, respectively, reach secular equilibrium within approximately 7.5 min after elution. Figure 16-10 shows a time-activity curve demonstrating secular equilibrium. The characteristics of radionuclide generator systems are compared in Table 16-2.
those for the Rb-82 generators: 0.02 µCi of Sr-85 per mCi of Rb-82 or (0.02 kBq/MBq). The Mo-99 contamination is evaluated by placing the Tc-99m eluate in a thick (˜6 mm) lead container (provided by the dose calibrator manufacturer), which is placed in the dose calibrator. The high-energy photons of Mo-99 can be detected, whereas virtually all of the Tc-99m 140-keV photons are attenuated by the lead container. Eluates from moly generators rarely exceed permissible Mo-99 contamination limits. The quality control procedures to evaluate breakthrough of radionuclidic contaminates in the eluates from Mo-99/Tc-99m and Sr-82/Rb-82 generators are discussed further in Chapter 17 in the context of dose calibrator operations and quality control. It is also possible (although rare) for some of the alumina from the column to contaminate the Tc-99m eluate. Alumina interferes with the preparation of some radiopharmaceuticals (especially sulfur colloid and Tc-99m-labeled red blood cell preparations). The USP limits the amount of alumina to no more than 10 mg alumina per mL of Tc-99m eluate. Commercially available paper test strips and test standards are used to assay for alumina concentrations.
TABLE 16-2 CLINICALLY USED RADIONUCLIDE GENERATOR SYSTEMS IN NUCLEAR MEDICINE | ||||||||||||||||||||||||||||||||||||||||||||||||
---|---|---|---|---|---|---|---|---|---|---|---|---|---|---|---|---|---|---|---|---|---|---|---|---|---|---|---|---|---|---|---|---|---|---|---|---|---|---|---|---|---|---|---|---|---|---|---|---|
|
TABLE 16-3 PHYSICAL CHARACTERISTICS OF CLINICALLY USED RADIONUCLIDES | ||||||||||||||||||||||||||||||||||||||||||||||||||||||||||||||||||||||||||||||||||||||||||||||||||||||||||||||||||||||||||||||||||||||||||||||||||||||||||||||||||||||||||||||||||||||||||||||||||||||||||||||||||||||||||||||||||||||||||||||||||||||||||||||||||||||||||||||||||||||||||
---|---|---|---|---|---|---|---|---|---|---|---|---|---|---|---|---|---|---|---|---|---|---|---|---|---|---|---|---|---|---|---|---|---|---|---|---|---|---|---|---|---|---|---|---|---|---|---|---|---|---|---|---|---|---|---|---|---|---|---|---|---|---|---|---|---|---|---|---|---|---|---|---|---|---|---|---|---|---|---|---|---|---|---|---|---|---|---|---|---|---|---|---|---|---|---|---|---|---|---|---|---|---|---|---|---|---|---|---|---|---|---|---|---|---|---|---|---|---|---|---|---|---|---|---|---|---|---|---|---|---|---|---|---|---|---|---|---|---|---|---|---|---|---|---|---|---|---|---|---|---|---|---|---|---|---|---|---|---|---|---|---|---|---|---|---|---|---|---|---|---|---|---|---|---|---|---|---|---|---|---|---|---|---|---|---|---|---|---|---|---|---|---|---|---|---|---|---|---|---|---|---|---|---|---|---|---|---|---|---|---|---|---|---|---|---|---|---|---|---|---|---|---|---|---|---|---|---|---|---|---|---|---|---|---|---|---|---|---|---|---|---|---|---|---|---|---|---|---|---|---|---|---|---|---|---|---|---|---|---|---|---|---|---|---|---|---|---|---|---|---|---|---|---|---|---|---|---|---|---|---|---|---|
|
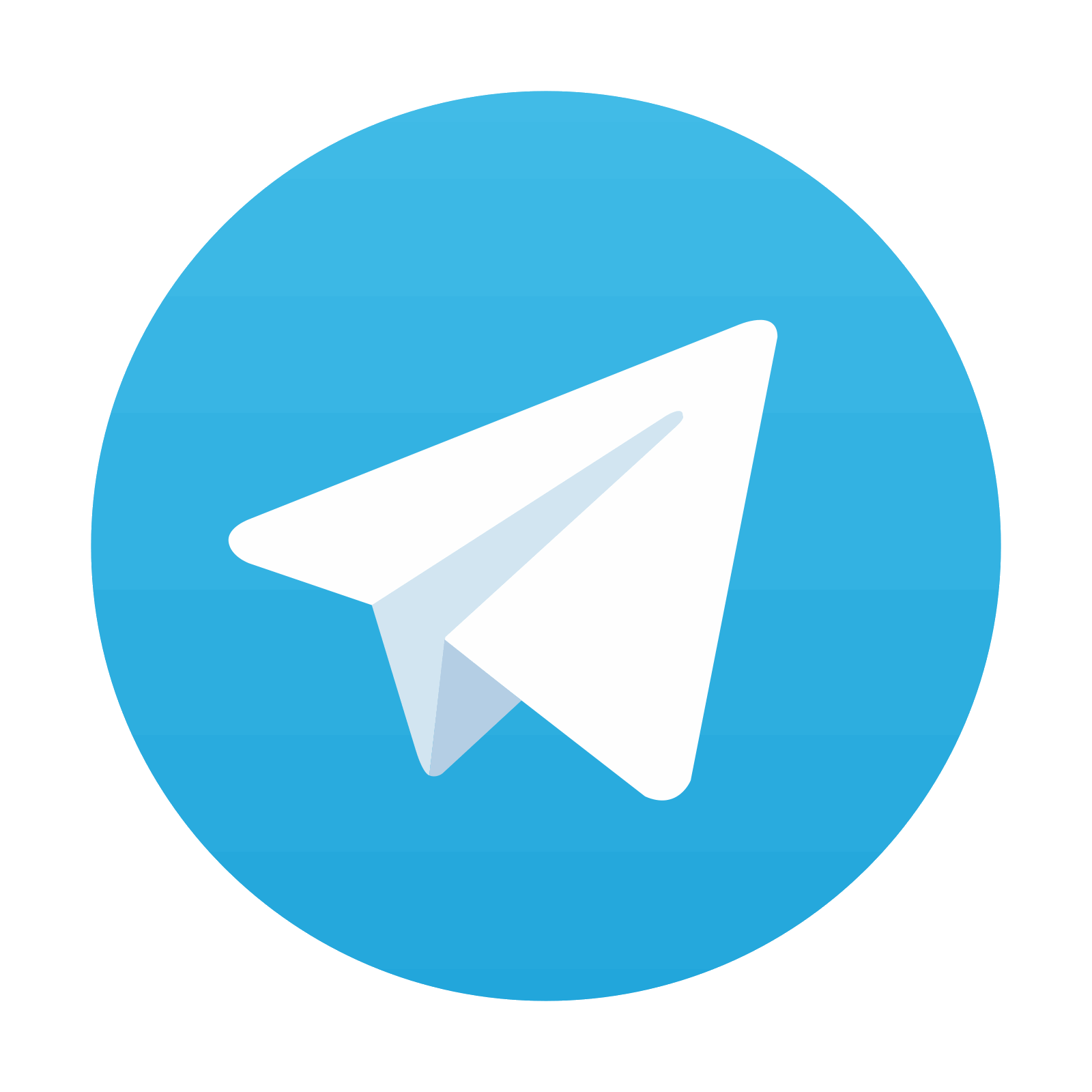
Stay updated, free articles. Join our Telegram channel
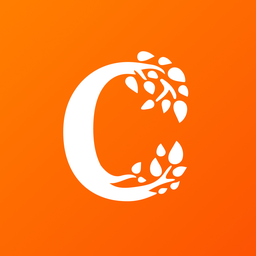
Full access? Get Clinical Tree
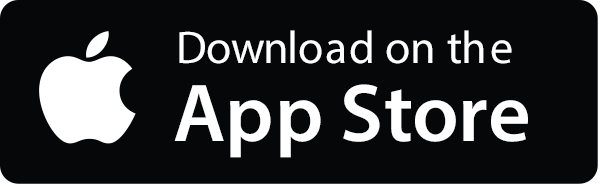
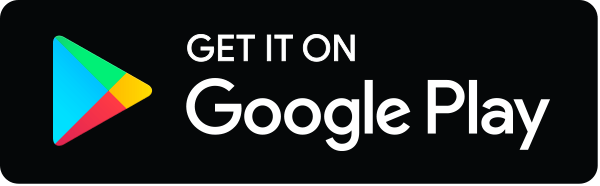