99mTc
99mTc-Bisphosphonates accumulate at sites of bone mineral rearrangement
99mTc-Labeled colloids are used for lymphoscintigraphy and imaging liver and spleen
99mTc-Hexakis-methoxy-isobutyl-isonitrile and 99mTc-tetrofosmin (a lipophilic cationic diphosphine) are used for myocardial perfusion imaging, identification of parathyroid adenoma, and identification of some tumors
123I or 131I
Iodide—localization of thyroid tissue
Metaiodobenzylguanidine (MIBG), a catecholamine analog, localizing in pheochromocytoma and neuroblastoma
201Tl
Ionic thallium used for tumor perfusion imaging
111In-Pentetreotide
Detects overexpression of somatostatin receptors, especially in neuroendocrine tumors and lesions arising from the neural crest, such as carcinoid, paragangliomas, and medullary thyroid carcinomas
111In-Capromab pendetide
Capromab pendetide is a murine monoclonal antibody which recognizes a transmembrane glycoprotein expressed by poorly differentiated and metastatic prostate adenocarcinomas
67Ga-Citrate
Gallium forms a complex with transferrin or lactoferrin. Receptors for this complex are overexpressed on membranes of tumor and inflammatory cells
Common single-photon radiopharmaceuticals for therapy
131I | For treatment of thyroid cancer and radioimmune therapy of lymphoma |
153Sm-EDTMP and 89SrCl | |
For treatment of intractable bone pain | |
90Y | For radioimmune therapy of lymphoma, radiopeptide therapy of neuroendocrine tumors, and complexed to resin particles or glass spheres for intra-arterial radioembolization therapy of hypervascularized tumors of the liver |
Common single-photon radiopharmaceuticals used for oncological diagnosis include the “so-called” technetiated radoipharmaceuticals such as 99mTc-bisphosphonates (that accumulate at sites of bone mineral rearrangement), 99mTc-Labeled colloids (that are used for lymphoscintigraphy and for imaging of the liver and spleen), 99mTc-Hexakis-methoxy-isobutyl-isonitrile and 99mTc-tetrofosmin (initially employed for myocardial perfusion imaging, then validatyed also for localization of parathyroid adenomas and for identification of other malignant tumors).
The most commonly used radiopharmaceuticals labelled with radioiodine (123I or 131I) include Iodide itself (for localization of thyroid tissue) and the catecholamine analog Metaiodobenzylguanidine (MIBG, for localizing pheochromocytoma and neuroblastoma). 201Tl Ionic thallium can be used for tumor perfusion imaging, while 111In-Pentetreotide detects overexpression of somatostatin receptors, especially in neuroendocrine tumors and in lesions arising from the neural crest, such as carcinoid, paragangliomas, and medullary thyroid carcinomas. 111In-Capromab Pendetide is a murine monoclonal antibody which recognizes a transmembrane glycoprotein expressed by poorly differentiated and metastatic prostate adenocarcinomas. 67Ga-Citrate receptors are overexpressed on membranes of both tumor and inflammatory cells. Common radionuclides used for radiometabolic therapy include 131I, 153Sm, 89Sr, 90Y and 177Lu. Therapeutic radiopharmaceuticals are employed for therapy of differentiated follicular thyroid carcinomas, for therapy of pheochromocytoma/paraganglioma/neuroblastomas, for bone pain palliation, for radioimmunotherapy of lymphomas, for peptide radioreceptor therapy of neuroendocrine tumors, and for intra-arterial radioembolization of hypervascularized tumors of the liver. Experimental radionuclides for therapy include 89Zr and for radioimmunotherapy and peptide radioreceptor therapy as well as alpha emitters, such as 223Ra, 211At, 212Bi, 213Bi, and 225Ac for treating intractable bone pain as well as for radioimmunotherapy.
Keywords
Single-photon emission imagingRadiopharmaceuticalRadionuclideRadioimmune therapyNuclear oncologyRadioisotopesBackground
A radiopharmaceutical is a compound containing a radionuclide for the detection, characterization, or treatment of disease. Fundamental properties commonly associated with radiopharmaceuticals are as follows: (1) they are administered in mass amounts that rarely elicit a pharmacologic response and (2) interaction of radiopharmaceuticals with physiologic systems is not linked to their physical properties (emission of ionizing radiation) but rather to their chemical nature.
The chemistry of some radiopharmaceuticals is very simple (such as the ionic form of the radionuclide itself). Most radiopharmaceuticals have a complex chemical structure in which the radionuclide only serves for emission of the physical sign (see below), while interaction with the physiological systems is due to the chemical structure of the main carrier molecule.
Radiopharmaceuticals employed for diagnostic purposes produce a physical signal (emission of γ-rays and/or beta particles (β− or β+) that radiation sensors can detect. The nuclear medicine equipment employed for imaging (scintigraphy) produces an image that represents the distribution of the radiopharmaceutical in the body (see Chaps. 3 and Chaps. 4).
Therapeutic radiopharmaceuticals usually emit electrically charged particles (usually β− or α++ particles) that deposit their energy in the tissue, causing radiation damage to the site of localization, typically killing the cell. The emitted particles travel a very short distance from their emission point (few millimeters, or even few micrometers). In addition to particle emission causing a cytocidal radiobiologic effect, some therapeutic radiopharmaceuticals also emit γ-rays, which can be detected and can provide information on their distribution in the body. This information confirms the concentration of the radiopharmaceutical in the tissue and allows calculation of the local radiation dose (see Chap. 6).
The diagnostic information provided by scintigraphic imaging (or the desired therapeutic effect) derives from the characteristic distribution within the body of any given radiopharmaceutical. Most radiopharmaceuticals are administered systemically (i.e., intravenously); less frequent modalities of administration include the oral, interstitial, intracavitary, or even the intra-arterial routes. The main parameters that determine the scintigraphic information are the rate of disappearance (or clearance) from blood, accumulation, retention, and washout at a specific tissue/organ of interest. Changes in the distribution reflect pathophysiological changes of function or metabolism. Many of the abnormalities that can be demonstrated using radiopharmaceuticals occur at an early stage of the pathogenetic cascade that characterizes a disease, often before modifications in shape, size, and/or structure of the tissue/organ of interest can be detected using anatomic imaging modalities.
Radionuclide therapy can be employed with a curative intent of a tumor (e.g., for differentiated thyroid cancer), for palliation purpose only (e.g., for pain from metastatic skeletal disease), or to reduce an organ’s function (e.g., for hyperthyroidism).
Radiopharmaceuticals consisting of only the radionuclide in its ionic form usually concentrate in specific target tissue/organs because they are either radioisotopes of a naturally occurring element of biologic interest (such as, e.g., 123I, 124I, or 131I undergoing the same physiologic distribution of native 127I) or because they share chemical similarities/analogies with native elements of biologic interest (such as, e.g., 201Tl or 82Rb mirroring the physiologic distribution of native 39K).
For more complex radiopharmaceuticals, the radionuclide is incorporated in the main carrier molecule through a chemical process denominated “radiolabeling.” The radionuclides most widely employed in nuclear medicine and their main physical characteristics are reported in Table 2.1. Figure 2.1 plots the maximum range in water (a parameter reflecting penetration in soft tissues) for the β− and the β+ particles as a function of their energy. Their average range of penetration in tissues corresponds to about one-third of the respective maximum ranges.
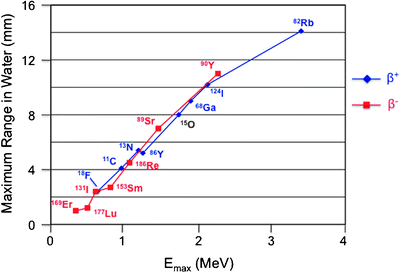
Table 2.1
Most widely used radionuclides in nuclear oncology and their main physical properties
Radionuclide | T 1/2 | Energy (keV) | Emission |
---|---|---|---|
123Ia | 12.8 h | 159 | γ |
Auger electrons | |||
131I | 196.8 h | 284, 364, 637 | γ |
810 | β− | ||
99mTc | 6.06 h | 142 | γ |
111Ina | 67.9 h | 172, 245 | γ |
Auger electrons | |||
67Gaa | 78.3 h | 93, 185, 296 | γ |
201Tla | 72 h | 135–167 | γ |
69–83 | x | ||
18F | 109.8 min | 634 | β+ |
11C | 20.4 min | 1,982 | β+ |
68Ga | 68.3 min | 770, 1,880 | β+ |
86Y | 14.8 h | 1,250 | β+ |
124I | 4.18 days | 603, 2,130 | β+, γ |
90 Y | 64.8 h | 2,270 | β− |
188Re | 16.8 h | 2,110 | β− |
155 | γ | ||
177Lu | 6.71 days | 500 | β− |
113, 208 | γ | ||
153Sm | 45.6 h | 800 | β− |
70, 103 | γ | ||
89Sr | 50.5 days | 1,460 | β− |
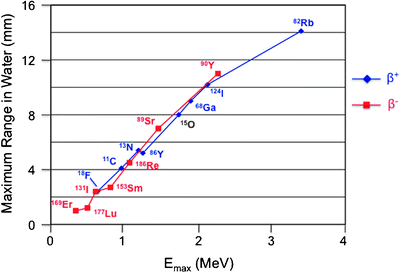
Fig. 2.1
Correlation between maximum energy (Emax, expressed in MeV) and maximum range of either β+ or β− particles emitted during decay of different radionuclides employed for either diagnostic purposes (β+) or therapeutic purposes (β−). Most of the energy deposited by each emission is nevertheless limited within a much shorter range (about 20% of the maximum range)
Radiopharmaceuticals can also be classified based on physical characteristics of the radionuclide (type and energy of the characteristic emissions, physical half-life) or else on their physicochemical properties of the molecule (size, hydro- or lipophilicity, positive, negative, or neutral electrical charge) and mechanism of localization (Table 2.2).
Table 2.2
Main mechanisms of in vivo distribution/localization of radiopharmaceuticals
Mechanism(s) | Rationale | Example |
---|---|---|
Mechanical trapping (capillary blockade) | The regional distribution of perfusion in an organ can be assessed by administering in the afferent blood vessel radiolabeled particles that, because of their large size (tens of μm), cause microembolization in the precapillary bed of an organ | 99mTc-MAA for lung perfusion scintigraphy (intravenous) |
90Y-Microspheres for treatment of liver tumors (intra-arterial) | ||
Phagocytosis | Upon interstitial administration, micellar-size radiopharmaceuticals are drained through the lymphatic system and are engulfed by macrophages lining the sinusoidal spaces of lymph nodes | 99mTc-Radiocolloid for lymphoscintigraphy and radioguided sentinel lymph node biopsy |
Ion exchange (chemisorption) | Exchange of ions between two electrolytes or between an electrolyte solution and a complex (binding to hydroxyapatite crystals or to amorphous calcium phosphate) | 99mTc-Labeled bisphosphonates for bone scintigraphy |
Membrane transport: simple, facilitated, and active transport | Passive transmembrane diffusion of lipophilic, cationic molecules that then bind to cytosolic components or accumulate into mitochondria | 99mTc-Tetrofosmin or 99mTc-sestamibi |
Facilitated diffusion mediated by specific transport proteins: glucose transporter (GLUT 1–5) expressed on the cell membrane as a function of energy requirements | [18F]FDG for PET of tumors with high glucose consumption | |
Active, energy-dependent transmembrane transport of molecules against a concentration gradient: sodium–iodide symporter | Radioiodine and 99mTc-pertechnetate for thyroid scintigraphy | |
Enzyme-mediated intracellular trapping | Trapping into cells as the result of a specific interaction of the radiopharmaceutical with an enzyme: phosphorylated [18F]FDG not being the substrate for subsequent metabolic pathways | [18F]FDG for PET of tumors with high glucose consumption |
Competitive substrates | Radiopharmaceutical competes with the endogenous substrate for the same sites at the catalytic enzyme: [18F]FLT, a thymidine analog, competes with native thymidine incorporation into DNA during replication | [18F]FLT PET for tumors with high proliferation rate |
Receptor-mediated probes | The radiopharmaceutical competes with the physiologic ligands for binding with the target receptor | Somatostatin analogs used for neuroendocrine tumors as vehicles to guide radioactivity to tissues expressing somatostatin receptors |
Non-receptor-mediated binding | Radiolabeled antibodies (whole antibodies or engineered minibodies) targeting specific antigen sites on the surface of tumor cells | 90Y-Ibritumomab tiuxetan (anti-CD20) for radioimmunotherapy of non-Hodgkin’s lymphoma (NHL) |
Single-Photon Emitting Radiopharmaceuticals Currently Used in Nuclear Oncology
99mTc-Labeled Radiopharmaceuticals
Because of its favorable gamma energy and lack of particulate radiation, 99mTc (a transition metal radionuclide) is employed for about 85% of single-photon imaging procedures performed in nuclear medicine. The eluate from the 99Mo/99mTc generator contains pertechnetate anion ([99mTcO4]−); this coordination compound has a very compact structure with tetrahedral geometry and oxidation state +7. Pertechnetate, the highest possible oxidation state for this metal, is also a very stable chemical species in aqueous solution. To use [99mTcO4]− to label a given molecule, it is necessary to remove the oxygen atoms bound to the metal through a reducing agent and to replace them with new ligand-coordinated atoms. During this process, the oxidation state of technetium is reduced to values lower than +7. The stannous ion (Sn2+) is generally used as a reducing agent, being added in aqueous solution as a chloride salt (SnCl2) in such small amounts that it does not alter solubility of the preparation or cause toxicity to the patient. Removal of the oxygen atoms from [99mTcO4]− occurs through the formation of Sn(OH)4 and other similar compounds. The atom of technetium is now free to coordinate with a binder (or chelating agent, such as DTPA) that has been preattached to the molecule to be labeled. The binder must have a high technetium coordinating ability to ensure stability of new 99mTc-labeled radiopharmaceutical and prevent it from recombining with the oxygen atoms in aqueous solution and thus return back to the anion pertechnetate or form technetium dioxide (TcO2), a secondary compound of oxygen that tends to form colloidal particles. Other 99mTc-labeling reactions involve different chemical approaches, such as derivatization with 6-hydrazinopyridine-3-carboxylic acid (HYNIC) for cross-linking.
99mTc-Sodium Pertechnetate
99mTc-Sodium Pertechnetate (Na99mTcO4) is obtained when eluting a 99Mo/99mTc generator with saline. 99mTc-Sodium Pertechnetate can be used as a radiopharmaceutical by itself. After i.v. administration, the pertechnetate ions circulate in the blood partly in their free form and partly bound to plasma proteins; because of their small size, free ions leave easily the vascular compartment and migrate to the interstitial fluids. As their concentration in blood diminishes because of migration to the extravascular space, other [99mTcO4]− ions are released from their binding to plasma proteins. Therefore, visualization of the vascular space observed in early scintigraphic acquisitions decreases gradually over time.
Pertechnetate ions are concentrated in tissues with anion pump activity, such as the thyroid, stomach, salivary glands, intestine, choroid plexus, and kidney.
Accumulation of [99mTcO4]− in thyroid follicular cells is mediated by the sodium–iodide symporter (NIS), a transmembrane protein consisting of 643 amino acids arranged in 13 domains with extracellular amino-terminal and intracellular carboxy-terminal (molecular weight ranging between 79 and 90 kDa, depending on the degree of glycosylation). NIS is located on the basolateral membrane of thyroid cells, and it is able to transport simultaneously sodium and iodide (with a stoichiometric ratio of 2:1) from the extracellular space within the thyroid cells. Expression of this protein is regulated, among other minor mechanisms, by the TSH, Thyroid Stimulating Hormone (upregulation) and by the concentration of iodide in extracellular fluids (downregulation) and is encoded by a gene on chromosome 19p12–13.2. The extraction of iodine from plasma and its concentration in thyroid cells is a saturable and active process that takes place against an electrochemical gradient (the intracellular concentration of iodine is 20–40 times higher than in plasma) and requires energy which is ensured by the Na-/K-dependent ATPase system.
The pertechnetate ion is transported by NIS from the extracellular to the intracellular compartment because it mimics the iodide ion, to which 99mTcO4− is similar in terms of mass, size, and charge density; however, once inside the thyroid cell, 99mTcO4− does not undergo subsequent organification as iodide does to participate in the synthesis of thyroid hormones.
In nuclear oncology, 99mTcO4− is used in patients with nodular goiter to define a nodule as either “hot” (increased uptake of the radiopharmaceutical relative to normal parenchyma) or “cold” (decreased uptake). Hot nodules correspond to hyperfunctioning tissue and are very rarely malignant. Cold nodules correspond to thyroid tissue with reduced function, and they can harbor malignancy. Differential diagnosis of a cold nodule as benign or malignant can only be made with fine-needle aspiration cytology. As mentioned above, the prevalence of thyroid cancer in hot nodules is very low (between 0% and 4% in adults), while up to 20% of the cold nodules are malignant.
99mTc-Diphosphonates
Virtually all radiopharmaceuticals employed for bone scintigraphy exhibit a similar pattern of distribution, characterized by accumulation in the bone linked to deposition of the mineral component. Early investigations on bone metabolism employed radionuclides with unfavorable physical properties. Agents such as 32P (in the chemical form of orthophosphate), radioactive calcium (45Ca, 47Ca) or calcium analogs (85Sr, 87mSr) in ionic form, and 18F-fluoride were used for evaluating the rate of bone turnover. Although images were obtained with 85Sr, it was only after the introduction of 99mTc-labeled phosphate agents (including pyrophosphate, polyphosphates, and phosphonates) that bone scintigraphy actually became one of the most frequently performed diagnostic nuclear medicine procedures.
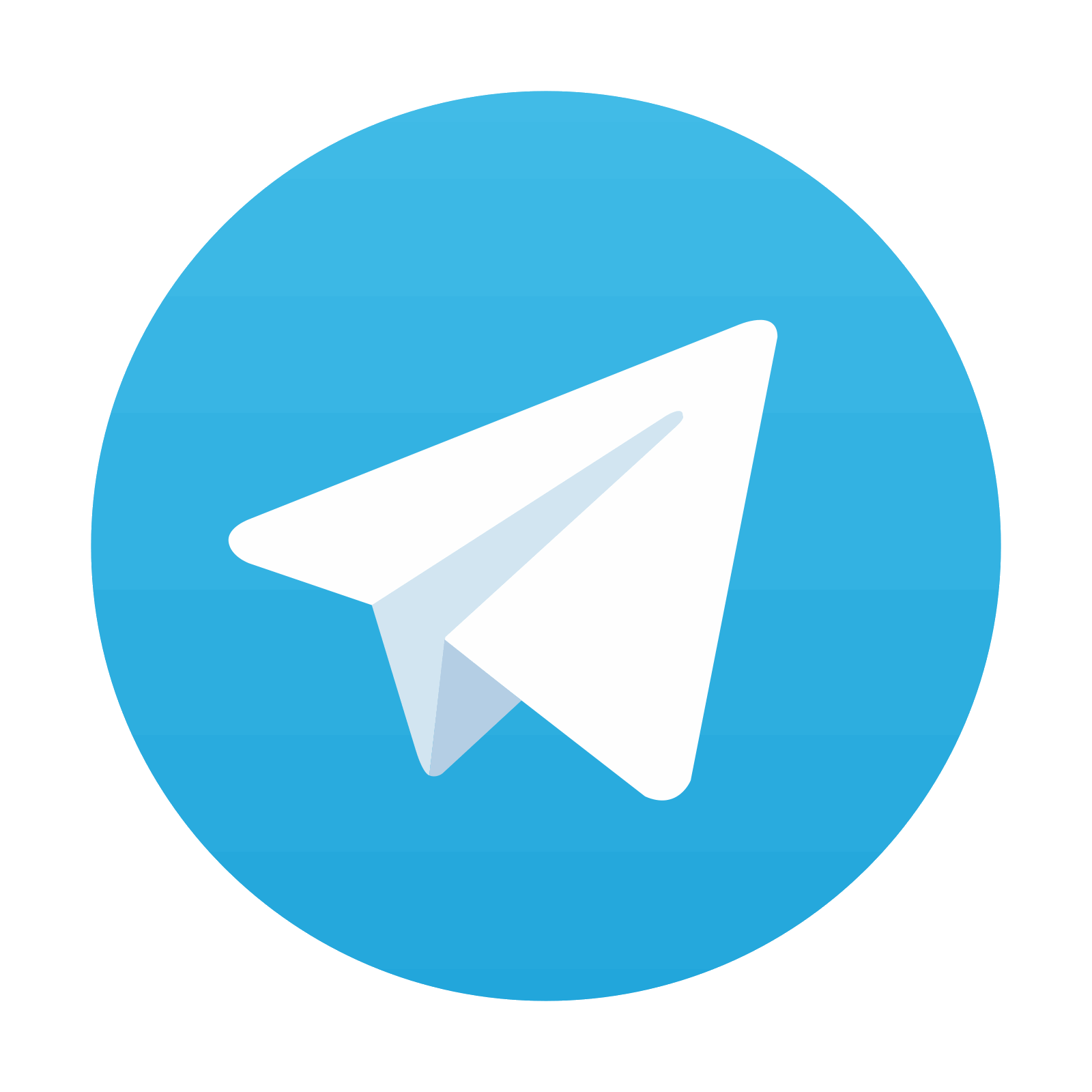
Stay updated, free articles. Join our Telegram channel
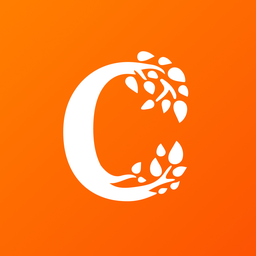
Full access? Get Clinical Tree
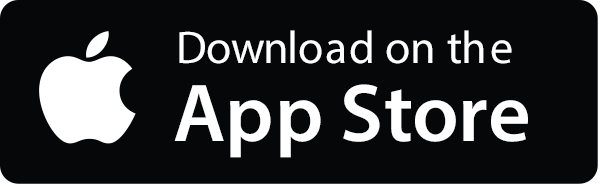
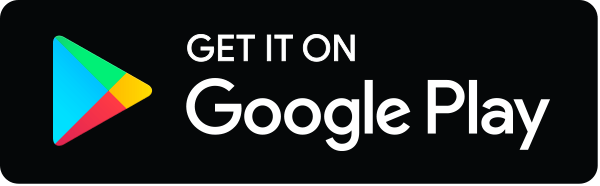
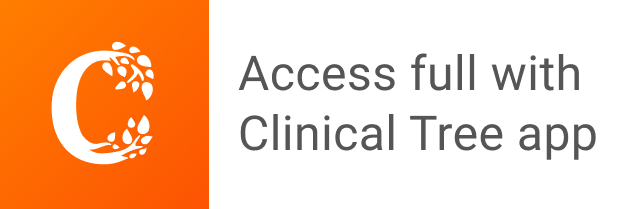