(1)
Manchester Institute of Biotechnology, University of Manchester, Manchester, UK
Abstract
Secondary ion mass spectrometry (SIMS) is capable of providing detailed atomic and molecular characterization of the surface chemistry of (bio)molecular samples. It is one of a range of mass spectrometry imaging techniques that combine the high sensitivity and specificity of mass spectrometry with the capability to view the distribution of analytes within solid samples. The technique is particularly suited to the detection and imaging of small molecules such as lipids and other metabolites. A limit of detection in the ppm range and spatial resolution <1 μm can be obtained. Recent progress in instrumental developments, including new cluster ion beams, the implementation of tandem mass spectrometry (MS/MS), and the application of multivariate data analysis protocols promise further advances. This chapter presents a brief overview of the technique and methodology of SIMS using exemplar studies of biological cells and tissue.
Key words
ToF-SIMSImagingMass spectrometryCellular analysisTissue analysisMolecular depth profiling3D SIMS1 Introduction
Secondary ion mass spectrometry (SIMS) is a technique in which material desorbed from a surface by keV ion bombardment is analyzed by mass spectrometry [1]. Originally developed in the 1950s and 1960s by Herzog et al. and Honig et al. to analyze metals and oxides, the technique has matured into a powerful and versatile analytical tool. Various operational modes have arisen from the development of the instrumentation and improved understanding of the underlying physics. Molecular SIMS for surface analysis was first demonstrated by Benninghoven in 1970 [2].
Alongside other techniques for mass spectrometry imaging, SIMS shows considerable promise in many areas of biological and biomedical research, including single-cell and tissue imaging. SIMS, unlike many techniques for biological imaging/analysis, does not require derivatization/labelling of the compounds to be detected. Other advantages of the technique are its high sensitivity and specificity, particularly to small molecules such as drugs and metabolites, and its high lateral resolution. Applications now include a wide variety from the life sciences, including molecular biology and medicine. This chapter briefly describes the basic theory of the SIMS technique and details the methodology for molecular imaging and analysis of biological materials using examples from the literature. For a more comprehensive discussion of the application of SIMS and related techniques, in these areas the reader is directed towards a number of recent review articles [3–6].
1.1 Secondary Ion Generation
The impinging primary ions deposit their energy in the surface and subsurface of the sample, typically through collision cascades in sample atoms and molecules. Where these cascades return to the surface, secondary particles (electrons and both atomic and molecular ions/neutrals) can escape surface binding energies and be ejected or sputtered. Secondary ions are extracted into a mass spectrometer, typically a time-of-flight (ToF) design to maximize transmission, where their mass-to-charge ratio m/z is determined and they are detected. Only species in the top few atomic/molecular layers (~1–10 nm) of the sample are sputtered leading to a surface mass spectrum. The secondary ion flux sputtered from a molecular sample consists of atomic, molecular, and fragment ions. The number of secondary particles sputtered per incident primary ion is termed the sputter yield. For organic materials the ionization probability of molecular secondary species is typically 10−3–10−7. Together these parameters lead to the secondary ion yield, which is a key feature in determining the sensitivity of the technique.
Secondary ion yields are influenced by the local chemical environment of the sputtered material. For atomic ions and inorganic samples, these matrix effects can result in secondary ion yield variations of several orders of magnitude for the same atom in different chemical environments. Quantitative analysis of these ion signals requires calibration using standard reference materials. In organic systems the effects are generally less severe and with careful experimentation relative concentrations of similar analytes in similar matrices can be determined.
The nature of the sputtering process means that the SIMS technique is inherently destructive on the microscopic scale. The progressive removal of material and exposure of the subsurface to the primary beam lead to the possibility of depth profiling, the analysis of successively deeper regions of the sample. This approach is known as dynamic SIMS, a powerful and well-established tool for elemental analysis of heterogeneous inorganic materials such as semiconductor devices [7]. Elemental analysis by the dynamic SIMS technique now encompasses biological analysis using a method of stable isotope monitoring [8]. Traditionally, the analysis of the molecular chemistry of the sample has been limited to the surface layer due to the disruption of chemical bonds resulting from the penetration of keV atomic primary ions 10s of nm into the bulk sample. To ensure that the secondary ions reflect the undamaged surface, each primary ion must impinge on a new area of the sample. This leads to static SIMS (SSIMS) analysis. Statistically this means that <1 % of the sample can be impacted by primary ions to ensure static conditions. The surface density of atoms is typically 1015 atoms cm−2 so the so-called static limit equates to a primary ion fluence of 1013 ions cm−2. The advent of polyatomic primary ion sources has (for many molecular materials) removed the constraints of the static limit, facilitating molecular depth profiling [9, 10] and 3D molecular imaging [11]. These exciting new capabilities are discussed below in the context of biological analysis.
1.2 Mass Spectrometry
Magnetic sector, quadrupole, and time-of-flight (ToF) mass analyzers have all been used in SIMS. Each spectrometer has specific advantages and disadvantages. In static SIMS the ToF-MS has become dominant, largely due to its very high transmission (>50 %) and parallel detection of all masses. These features mean that ToF-SIMS instruments exhibit very high sensitivities (ppm-ppb detection limits), which are necessary to achieve analysis under static conditions or where the amount analyte available is limited, e.g., in imaging applications where the total number of molecules in a single pixel can limit the available spatial resolution. Generally, ToF-MS requires that the source of analyte ions be temporally pulsed. Mass resolution is limited by the precision with which the extraction time of the analyte ions is defined. In ToF-SIMS the primary ion beam is pulsed by electrostatic deflectors, producing ~10–100 ns primary pulses. These can subsequently be bunched to <1 ns, increasing mass resolution without loss in sensitivity. Producing such short ion beam pulses reduces the lateral resolving power due to the variation in kinetic energy the ions acquire during the bunching process, and a compromise has to be met depending on the demands of the analysis. In ToF-SIMS mass resolution is also dependent on the inherent energy spread (10–100 eV) of secondary ions caused by the sputtering process. This energy distribution can be compensated for by an energy analyzer in the flight tube. The most common device is an ion mirror or reflectron through which ions of the same m/z but different kinetic energy experience different path lengths, bringing them back into temporal focus at the detector [12]. ToF-SIMS instruments typically include a sample entry system, a preparation chamber which might include a sample store or freeze-fracture device, a cold stage for holding the sample during analysis, optical viewing, one or more primary ion sources, a low-energy electron source, mass spectrometer, and ion and secondary electron detectors (see Fig. 1). Recently, alternative instrumental configurations have been explored to exploit the properties of “low damage” polyatomic sputtering and to facilitate enhanced molecular depth profiling and 3D imaging by ToF-SIMS. Winograd and co-workers adapted a commercial hybrid quadrupole-ToF-MS instrument based on orthogonal extraction to allow high mass resolution data (m/Δm >10,000) to be acquired through continuous C60 + sputtering [13]. This allowed increased data rates and removed the necessity of fast-pulsing the primary beam which degrades the spot size. Vickerman and co-workers have developed a new instrument based on a similar principle, decoupling the sputtering from the ToF-MS measurement, in this case using a linear buncher to compress secondary ions into a time focus at the entrance to the ToF-MS [14]. This design allows almost continuous analysis of sputtered ions from a primary beam working in DC mode. Heeren and co-workers have added a C60 +SIMS source to a FT-ICR MS, providing mass resolution >100,000 [15]. Image acquisition rate in ion trap instruments such as the FT-ICR MS is however limited by the detection time, currently ~1 pixel per second. These various instrumentation developments also permit MS/MS analysis for enhanced structure determination of detected ions, a feature extremely useful in biological analysis, but one that very seldom has been available in SIMS measurements.
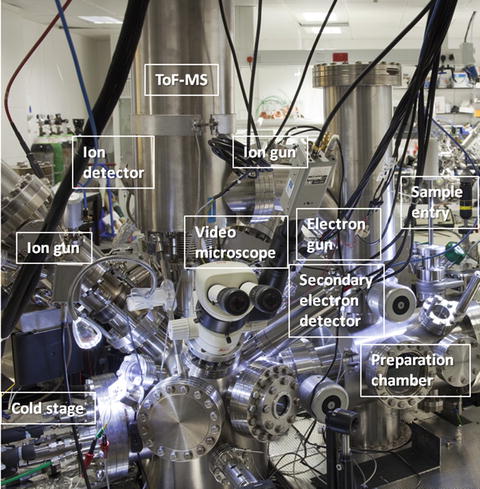
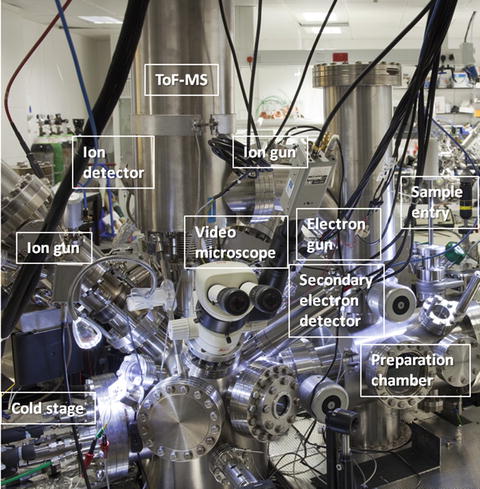
Fig. 1
A ToF-SIMS instrument of the standard reflectron design (BioToF-SIMS, Kore Technology, UK)
2 Materials
2.1 Specimen Preparation and Handling
1.
Substrate materials, e.g., polished Si (100) wafer 99.999 % (Advent Research Materials Ltd, Oxford, UK), indium-tin-oxide (ITO)-coated glass slides (4–8 Ω resistance, Delta Technologies, Stillwater, MN) (see Note 1).
2.
Ultrasonic bath for cleaning substrates and tools.
3.
HPLC grade methylene chloride, hexane, acetone, methanol.
CAUTION: flammable/toxic solvents.
4.
Ammonium formate (150 mM in DI water, pH 7.2–7.3).
5.
Glass pipettes for rinsing samples.
6.
Polystyrene “spacer” microspheres (5–50 μm, 1:1 volume mixture) (Duke Scientific, Palo Alto, CA).
7.
Aluminum kitchen foil.
8.
Hydrocarbon cryogen, e.g., propane or isopentane. CAUTION: highly flammable, explosion risk.
9.
Plunge-freezing machine, homemade or purchased, located in a fume hood or well-ventilated laboratory, and isolated from sources of ignition.
10.
Liquid nitrogen. CAUTION: cryogenic liquid.
11.
Cryo-storage vials (Nalgene system 100, Nalge Co., Rochester, NY).
12.
Embedding material for tissue cryosectioning, e.g., 10 % gelatin.
13.
Mounting material for tissue cryosectioning, e.g., Tissue-Tek (Sakura Finetek, Tokyo, Japan).
15.
Clean (vacuum) dessicator for storage of freeze-dried samples.
16.
Cryo-transfer sample stub, ideally with integral thermocouple.
17.
Nitrogen gas cylinder (5–10 bar) for flowing through ToF-SIMS cold stage heat exchangers. CAUTION: Asphyxiant.
2.2 ToF-SIMS Acquisition
1.
Imaging ToF-SIMS instrument in vibrationally isolated laboratory.
3.
Picoammeter or electrometer for measuring primary ion/electron dose (Model 485 Keithley Instruments Ltd, Theale, UK, www.keithley.com).
4.
A suitable “test” sample consisting of various specimens for tuning and measuring instrumental parameters such as sensitivity, mass resolution, and spatial resolution (see Note 3).
5.
Provision for computer control of primary ion beam raster and position.
6.
Optical microscope and/or CCD camera for viewing sample in analysis position.
3 Methods
This section describes the typical methodology required to achieve a successful ToF-SIMS analysis of a biological cell or tissue sample (see Note 6). As always, tools and substrates must be cleaned prior to use.
3.1 Specimen Preparation and Handling for Biological Cells and Tissue
Due to the ultrahigh vacuum (<10−8 mbar) requirements and extreme surface sensitivity of the technique, appropriate sample preparation and handling is essential. The aim of a successful sample preparation for ToF-SIMS is to preserve the native chemical state of the sample in a form that is compatible with the analysis. Where imaging is to be performed, it is particularly important to minimize the migration of diffusible ions. Endogenous species are often present in low concentrations in cells and tissue, so sensitivity is a key issue, particularly in imaging applications due to the limited amount of analyte per pixel. Sample preparation methods as well as improved instrumentation have been developed to enhance sensitivity.
Preparation of biological samples for ToF-SIMS predominantly involves cryofixation methods adopted from the cryo-electron microscopy community [16]. Cryogenic specimen preparation was first applied in the SIMS field for dynamic SIMS ion microscopy of elemental species such as isotopic tags [17]. The subject of sample preparation in mass spectrometry imaging has recently been reviewed by Goodwin [18] and by Kaletas et al. [19]. Particular attention here is drawn to the measures necessary to facilitate meaningful chemical imaging of small molecules and diffusible ions in biological material.
Malm et al. compared the preparation of human fibroblast (hTERT) cells on silicon for ToF-SIMS by the following means: (a) cryofixation followed by freeze-drying, (b) glutaraldehyde (GA, 2.5 % v/v in phosphate-buffered saline (PBS)) fixation followed by cryofixation and freeze-drying, and (c) GA fixation followed by alcohol drying (substitution of water with ethanol followed by removal of ethanol by desiccation or critical-point drying) [20]. The overall conclusions of this detailed study were that cryofixation and freeze-drying better retained intact membranes and the distribution of diffusible ions, whereas cell membranes were disrupted following GA fixation, and alcohol drying extracted lipid components.
Fletcher et al. compared 3D ToF-SIMS analysis of formalin-fixed (4 %, for 15 min at 4 °C), freeze-dried, and freeze-fractured frozen HeLa cells using a C60 + ion beam [21]. The authors concluded that cells maintained in a frozen hydrated state (<150 K) during the analysis showed reduced chemical redistribution, cleaner spectra, and improved chemical contrast in both 2D and 3D imaging (see Fig. 2).
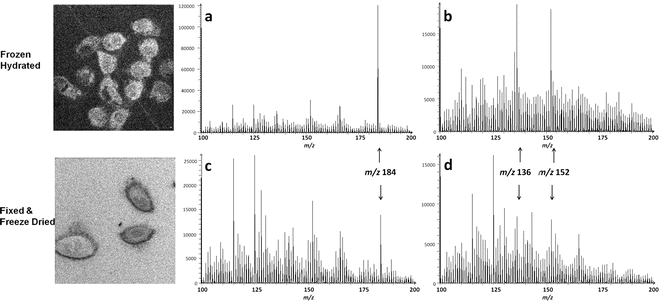
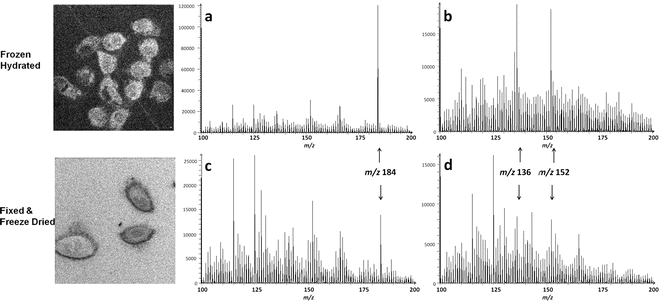
Fig. 2
ToF-SIMS analysis of HeLa cells prepared by (top row) cryofixation and analyzed in the frozen state and (bottom row) formalin fixation. Total ion images and regions of the positive ion SIMS spectra are shown at a depth corresponding to exposure of the cell nucleus (accumulated C60 +fluence of 6 × 1013 ions/cm2) (a) and (c) spectra from pixels surrounding the nucleus (b) and (d) spectra taken from pixels within the nucleus. The spectra (a, b) from the frozen sample are dominated by phosphocholine lipid headgroup m/z 184.1, m/z 136.1, and 152.1 corresponding to the protonated molecular ions of adenine and guanine, respectively. These are less abundant in the spectra (c and d) from fixed, freeze-dried cells. Data was acquired over a 250 × 250 μm2 field of view, 256 × 256 pixels, using a 40 keV C60 + beam. The images were reproduced from Fletcher et al. [21], with the permission of John Wiley & Sons, Ltd
In a further example, Wu et al. demonstrated that tissue types in a formalin-fixed, paraffin-embedded, and deparaffinized mouse embryo section could be differentiated using ToF-SIMS [22]. However, the basis of this discrimination was very low mass signals (mostly hydrocarbons and elemental species m/z<60), and this discussion will focus on the methods for preserving more molecular chemistry.
3.1.1 Specimen Mounting Procedures
Adherent cells can be grown on SIMS-compatible substrates, e.g., silicon wafer for ease of handling (see Note 7) [23]. Substrates should be cleaned and sterilized prior to cell culture, e.g., using 100 % ethanol and UV irradiation. Chandra et al. concluded that cells grown on silicon showed comparable growth rate and morphology to those grown on glass slides [24]. Cells cultured in suspension can be deposited on the substrate and the extracellular medium removed using a blotting or cytospin process [25]. It is important to remove buffer salts which would otherwise form a crust over the sample under vacuum and obscure the surface of interest.
Tissue samples are generally prepared by producing a 10–20 μm-thick section in a cryomicrotome (−16 to −26 °C is a typically cutting temperature) and then thaw-mounting the cryosection onto a SIMS substrate. Certain compounds, including optimal cutting temperature (OCT) polymers such as Tissue-Tek, commonly used by histologists for embedding small samples for cryosection are easily smeared across the section surface and known to induce ion suppression effects in mass spectrometry imaging [26]. This situation must be avoided and necessitates rigorous cleaning of the microtome after use with these materials. Ideally samples for SIMS analysis should not be embedded in a polymer containing cutting matrix, but rather should be adhered to the cutting block using a small amount of such material on the back face of the sample. If embedding is necessary, e.g., to preserve the morphology of the sample during cutting, then 10 % gelatin or agarose is preferable. The optimum thickness of the cryosection will depend on the cutting temperature and the type of tissue. Thinner sections are less likely to provide charging problems under ion bombardment, whereas thicker sections are more mechanically robust and less likely to tear during handling. The latter point may be particularly important when cross-comparing SIMS data with images obtained on serial sections, e.g., by histology or other chemical imaging techniques. To allow direct optical microscopy on the SIMS sample, transparent substrates should be used. Standard microscope glass is electrically insulating and requires careful charge compensation in the SIMS experiment; therefore it is preferable to use conducting indium-tin-oxide (ITO)-coated glass in this instance. Following cryosectioning and mounting the tissue slice can be dried at room temperature in a vacuum desiccator or freeze-dried prior to SIMS analysis.
3.1.2 Specimen Rinsing
Physiological fluids and buffer salts can obscure the chemical distribution of the underlying solid sample and introduce ion suppression effects that limit analyte sensitivity. Additionally, the presence of salts may prevent a sustained molecular ion signal during depth profiling [27, 28]. A number of studies have been performed to evaluate the effectiveness of removing these effects by rinsing the sample in various solutions [20, 29]. One method that seems generally applicable to cultured cells is to rinse with ammonium formate (150 mM in DI water, pH 7.2–7.3). Washing solutions should be iso-osmotic with the cellular medium preserving the osmotic pressure of the cell environment and while replacing the buffer with a medium volatile enough to evaporate leaving the surface relatively free from involatile buffer salts. The washing solution is dispensed onto the inclined sample using a clean glass syringe to avoid redepositing salts on the analyzed area. Alternatively the sample can be dipped briefly (~10 s) into a small beaker containing fresh washing solution. Samples are then dried under an inert gas flow. If rinsing is performed it is usually done so immediately prior to cryogenic processing. For cells that are destined for freeze-fracture, rinsing may be less beneficial, as a clean surface will be exposed by the fracture process. However, removal of extracellular matrix and nonvolatile buffer salts from the surrounding region will aid identification of the cellular region and provide a less complex chemical background in the resulting mass spectrum. Berman et al. have compared several rinsing solutions on cells that are then air-dried rather than fast-frozen [30]. The study concludes that ammonium formate is generally the most successful rinsing solution but diffusible ions subsequently leak out of the cytosol without the fast-freezing step. In developing a rinsing protocol for a particular tissue type and analyte(s) of interest, one should take care to check that analyte diffusion has not occurred, by analyzing an unwashed sample or by confirming distribution by another means, e.g., histology.
3.1.3 Promoting Analyte Ionization Through Sample Preparation
Various methods involving matrix addition have been reported to enhance the molecular sensitivity and spatial resolution in SIMS. The enhanced cationization of (bio)organic species in combination with metal salts or by deposition of a sub-monolayer of gold has been reported by Delcorte et al. [31, 32]. Wu and Odom mixed MALDI matrices such as 2,5,-dihydroxybenzoic acid (DHB) with peptides to enhance protonation and sensitivity, particularly at high mass [33]. This methodology, termed matrix-enhanced SIMS (ME-SIMS), was adapted to retain imaging capability by Altelaar et al. achieving μm resolution molecular images of cryosections of the cerebral ganglia of the freshwater snail Lymnaea stagnalis by depositing a thin layer of DHB on the nerve tissue [34]. Subsequently new, acidified matrices have been produced specifically for proton-donation purposes in ME-SIMS applications [35]. Sjövall and co-workers have been promoting cationization by imprinting freeze-dried cells onto a silver substrate [29, 36] or depositing a thin layer of silver onto cells [37] or tissue [38]. While these methods show considerable promise, they should be used with caution in imaging applications to ensure that the native analyte distribution is not perturbed.
3.1.4 Plunge-Freezing
Cells grown or deposited on SIMS substrates and tissue biopsies for subsequent cryosectioning can be prepared for ToF-SIMS by plunge-freezing to fix the distribution of analytes without resorting to chemical additives. The cryo-preparation methodology has been described in detail elsewhere in this volume and a brief summary only is presented here.
1.
Prepare a dewar (~100 mL) of freezing medium (e.g., liquid propane or other cryogen) in a fume cupboard to ensure good ventilation and minimize condensation.
CAUTION: Flammable liquids/gases—avoid sources of ignition.
2.
Prepare a storage dewar of LN2.
3.
For cell cultures, prepare the cells on a suitable substrate. If the specimen is to be fractured, add polystyrene “spacer” microspheres (1:1 vol. mixture) to avoid crushing the specimen, and place a second substrate on top, forming a “sandwich” structure.
4.
For tissue biopsies the samples can be placed in an aluminum foil basket.
5.
Hold the specimen substrate(s) in an automated plunge-freezing device or in suitable tweezers taking precautions to avoid touching the specimen itself (see Note 8). CAUTION: Cryogenic liquids—avoid skin contact.
6.
Plunge the specimen rapidly into the freezing medium.
7.
While under the cryogenic liquid and using precooled tweezers, place the specimen substrate into a cryovial. If using a single substrate, take care to avoid contaminating the specimen surface.
8.
Place the cryovial under LN2 in the storage dewar.
9.
Store specimen under LN2 until further processing or ToF-SIMS analysis.
10.
Cryosection the sample if required then dry in a desiccator or freeze-dry as described below.
3.1.5 Freeze-Drying
Freeze-drying of plunge-frozen specimens has proven successful in the preparation for ToF-SIMS of cell cultures and mounted tissue sections. The advantage of freeze-drying is that subsequent sample handling is simpler in that cryogenic temperatures do not need to be maintained (see Note 9). However, removal of the water from a biological sample will inevitably result in some relocation of biochemistry. Although this may be advantageous for detection, e.g., concentrating analytes that were dispersed in regions of high water content, interpretation of the 3D distribution of analytes in relation to the original, hydrated, sample should be performed with caution.
1.
Remove the frozen specimen from the LN2 storage dewar.
2.
If the sample is to be freeze-fractured, this should be performed at this stage, on the bench under LN2.
3.
Load the frozen specimen into the freeze-dryer.
5.
Once freeze-drying is complete the specimen can be removed and stored in a desiccator for several days prior to analysis.
3.1.6 Transfer to ToF-SIMS
1.
Isolate the fast entry vacuum lock from the remainder of the ToF-SIMS instrument and vent it to dry nitrogen.
2.
Insert the specimen, attached to the sample stub, into the fast entry lock and evacuate it to ~10−3 mbar. If the sample is frozen, then try to minimize the formation of frost on the sample stub or specimen in transit between LN2 and the instrument. This can be achieved by working rapidly in a low-humidity environment and by using a transfer assembly with a hood over the sample stub.
3.
Transfer the specimen to the preparation chamber and evacuate it to the base pressure of the analysis chamber (typically 10−9 mbar).
3.1.7 In Situ Freeze-Fracture
For analysis in the frozen state, samples prepared by the plunge-frozen “sandwich” method described above must be fractured in vacuo to avoid the condensation of atmospheric water and contamination that would impair a surface analysis measurement. Several bespoke devices have been developed in different laboratories to perform this challenging task. Issues such as precise control over the sample temperature during manipulation and fracture are important to optimize the process and provide reproducibility (see Note 11).
Winograd and Ewing and co-workers pioneered the adoption of fast-freezing and in situ freeze-fracture methodology in ToF-SIMS analysis of frozen hydrated specimens using a variety of liposomes and cellular systems [39–41]. ToF-SIMS imaging has been combined with bright-field and fluorescence microscopy to image frozen hydrated, freeze-fractured rat pheochromocytoma (PC12) cells [42, 43]. This integrated approach aids identification of the fracture plane and subsequent interpretation of the ToF-SIMS data. An example of the application of this freeze-fracture methodology is the study of membrane composition in mating Tetrahymena thermophila, a free-living ciliated protozoa, demonstrating the formation of lipid domains in response to cell-to-cell conjugation [44]. ToF-SIMS images of the paired cells are shown in Fig. 3. The continuous distribution of C5H9 + (from acyl chains in phospholipids) shows the cells are in contact. Phosphatidylcholine (PC) lamellar lipids (indicated by the PC headgroup ion m/z 184+) are depleted at the cell junction in contrast to the nonlamellar lipid 2-aminoethylphosphonolipid (2-AEP) (indicated by the headgroup ion m/z 126).
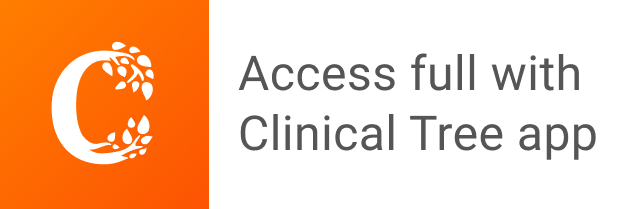