Fig. 22.1
Jablonski diagram outlining the photochemical processes involved in PDT
Upon absorption (ABS) of light energy by the sensitizer, an electron is promoted from the singlet ground state (S0) to a higher energy singlet excited state (S1, S2…). Any electrons that occupy orbitals higher than S1 return to S1 via a non-radiative process known as internal conversion. From S1 the electron has two options; (i) it can return to S0 emitting its excited energy as fluorescence (FLU), or (ii) undergo a process known as intersystem crossing (ISC), where the spin state of the excited electron is changed, forming a triplet excited state (T1). Triplet excited states have significantly longer lifetimes than singlet excited states, enabling T1 to partake in non-radiative processes kinetically unavailable to singlet excited states. In PDT, such processes are usually classified as Type I or Type II reactions. In Type I photoreactions, certain molecular substrates can be directly oxidized by the excited sensitizer, resulting in a reduced sensitizer that can further interact with molecular oxygen to generate superoxide ion (O2 −) and its derivative series of ROS (Macdonald and Dougherty 2001). In Type II photoreactions, the T1 state interacts directly with molecular oxygen, which itself has an uncharacteristic triplet ground state (T0), via an energy transfer process to produce singlet oxygen (1O2). While both Type I and Type II reactions can occur simultaneously, Type II reactions typically dominate in aerobic media. Due to the high reactivity and short half-life (0.04 μs) of singlet oxygen, its diffusion radius is less than 20 nm. This implies that only cells close to the site of its generation are affected (Moan and Berg 1991). Several factors influence the efficiency of ROS generation in PDT, such as the type of sensitizer used, its concentration, the light dose (both wavelength and fluence) and the amount of available oxygen. While PDT is extremely effective at treating superficial lesions and is a recognized first line treatment for non-melanoma skin cancer, its use for the treatment of deeper-seated solid tumors has been restricted by the inability of light to penetrate to depth through human tissue. The penetration of light through human tissue depends on its wavelength, with longer wavelength light penetrating deeper than shorter wavelength light. Indeed, the phototherapeutic window, where tissue has maximum transparency to light, is in the 750–900 nm range (Starkey et al. 2008). Unfortunately, the absorption maximum of currently approved sensitizers is in the 400–600 nm range, limiting penetration depth to millimeters. While significant progress has been made with the development of so-called third generation sensitizers, in particular the bacteriochlorins with strong absorption bands in the 720–740 nm region (Fukuzumi et al. 2008), the difficulty remains in delivering light to sites that are deep within the body.
22.2 Sonodynamic Therapy (SDT)
22.2.1 The Concept
The observation that relatively low intensity ultrasound can enhance chemotherapeutic drug action has been well documented by our own group and many others (Rosenthal et al. 2004; Tachibana et al. 2008; Li et al. 2008a, b; Nomikou et al. 2010). The mechanisms by which ultrasound enhances the action of chemotherapeutic drugs are varied and range from; (a) the ability of ultrasound to ‘porate’ cell membranes (a phenomenon known as sonoporation) to enhance entry of the chemotherapeutic drug into target cells to (b) the ability of ultrasound to promote dispersion of chemotherapeutic drugs through poorly vascularized tissues in solid tumors (Li et al. 2008a; Nomikou et al. 2010). Some have interpreted this as the drug sensitizing the tumor tissues since the approach reduces the effective dose of the chemotherapeutic drug that needs to be used to obtain a cytotoxic effect (Rosenthal et al. 2004). Thus in the field, some refer to this extremely broad interpretation as sonodynamic therapy. However, in 1989 it was recognized by Yumita and Umemura that hematoporphyrin, a well-known photosensitizer, could be used to elicit cytotoxic effects in acoustic fields. They then went on to coin the phrase ‘sonodynamic approach’ in 1992 to describe the activation of the porphyrin by ultrasound (Yumita et al. 1989; Umemura et al. 1992). It is now generally recognized that the term sonodynamic therapy refers more specifically to sensitizer-dependent sonochemical or sonophotochemical events in an acoustic field that lead to cytotoxicity. For the purposes of this review we will deal with this more narrow definition of SDT. Although we will review SDT in more detail below, the concept offers very significant advantage over alternative approaches involving the use of external stimuli, primarily because it exploits ultrasound at relatively low intensities. Essentially, the stimulus is non-thermal and as such is recognized as being non-toxic. In addition, with few exceptions, the approach exploits sensitizers that are commonly employed as photosensitizers in PDT, and these are also regarded as relatively non-toxic entities. Therefore, in comparison with the stimulus-dependent approaches described above, SDT offers the advantage of minimizing adverse effects and maximizing on-target responses. The approach also provides a very significant benefit over PDT because unlike light, ultrasound may be focused deeply within tissues to a single discrete point in three dimensions, whereas light diffuses very significantly as it passes through tissues and the depth to which it penetrates is extremely limited. Indeed, the latter is one of the major reasons why the use of PDT has been restricted to treatment of less accessible lesions. In addition, the use of a chemical agent that is non-toxic in the absence of the stimulus also adds another level of control and distinguishes the more narrow definition of SDT from the broader definition where ultrasound is employed to enhance the action of an already toxic chemical. In SDT, off-target effects associated with virtually all cancer chemotherapeutic drugs, even at lower doses, are circumvented.
22.2.2 Sonosensitizers
As mentioned above, many of the sensitizers originally used in SDT-based studies were porphyrin-based molecules that had been used as photosensitizers and these included hematoporphyrin and Photofrin®, a commercially available hematoporphyrin derivative that is employed in clinical PDT. Table 22.1 lists a selected group of sensitizers that have been studied extensively in the context of SDT. Even in the very early studies reporting sonodynamic effects, it was very clearly demonstrated that the porphryin-based sensitizers produced ROS when exposed to ultrasound and it was suggested that these ROS mediated ultrasound-responsive cytotoxicity in much the same way as in PDT. Indeed, it was demonstrated in a single study, using the same acoustic field properties, that protoporphryin IX appeared to be more active as a sonosensitizing agent than hematoporphyrin, although subsequent studies suggested that this was due to the observation that cells had simply taken up more protoporphryin IX (Liu et al. 2007; Zhu et al. 2010). Although porphyrins had been demonstrated to be capable of sonodynamic effects, it was recognized that these are relatively hydrophobic and although taken up preferentially by tumors to some degree, their distribution in other tissues is ubiquitous and clearance from those tissues is prolonged (Liu et al. 2007). If one wished to realize the major potential benefit offered by SDT, i.e. non-invasive access to deeper lesions, then it would be of benefit if the sensitizer did not exist in tissues anatomically positioned between the ultrasound source and the target.
Table 22.1
A selection of sensitizers used in SDT
Sensitizer | ROS | Ultrasound frequency | Reference |
---|---|---|---|
5-Aminolevulinic acid | N/A | 1.04 MHz | Ohmura et al. (2011) |
Acridine orange | 1O2 + ·OH | 2 MHz | Suzuki et al. (2007) |
ATX-70 | 1O2 + ·OH | 500 KHz | Ding et al. (2006) |
Chlorin-e6 | 1O2 + ROO | 1.56 MHz | Yumita et al. (2008) |
ClAl-phthalocyanine | N/A | 3 MHz | Yumita and Umemura (2004b) |
DCPH-P-Na(I) | 1O2 | 1 MHz | Hachimine et al. (2007) |
Hematoporphyrin | 1O2 | 1.92 MHz | Yumita et al. (1990) |
Hypocrellin SL052 | N/A | 1 MHz | Meng et al. (2010) |
Indocyanine green | N/A | 1 MHz | |
Methylene blue | ·OH | 2 MHz | Komori et al. (2009) |
Photofrin | 1O2 | 1 MHz | Xu et al. (2013) |
Phthalocyanine | N/A | 1 MHz | Kolarova et al. (2009) |
Protoporphyrin IX | 1O2 | 1 MHz | Guo et al. (2013) |
Rose Bengal | 1O2 | 0.8–5.9 MHz | Umemura et al. (1999) |
Rose Bengal derivative | 1O2 | 1.92 MHz | Sugita et al. (2010) |
On the other hand, others have reported the use of xanthene dyes, such as rose bengal, as sensitizers and demonstrated ultrasound-stimulated ROS generation in cell free systems and cytotoxic effects in–vitro (Kawabata and Umemura 1997; Umemura et al. 1999; McCaughan et al. 2011). Although extremely effective as a sonosensitizer in–vitro, its use is contraindicated in-vivo because of its extremely rapid sequestration in the liver and subsequent clearance (Sugita et al. 2007). Prompted by the latter and suggestions that for selective uptake of porphyrins by tumors, amphilicity was key, Sugita et al. (2007, 2010) sought to develop amphiphilic preparations of rose bengal using a combination of alkylation and carboxylation. The resulting drug, known as rose bengal derivative, was shown to be more effective as a sonosensitizing drug because it exhibited enhanced uptake by murine tumors (Sugita et al. 2007, 2010).
In addition to serving as sonosensitizers, the agents selected for Table 22.1 all exhibit the ability to serve as photosensitizers, with the exception of DCPH-P-Na (I) (13,17-bis(1-carboxyethyl)-8-[2,4 dichlorophenyl-hydrazonoethylidene]-e-ethenyl-7-hydroxy2,7,12,18-tetramethylchlorin, disodium salt). Synthesized from protoporphyrin IX dimethyl ester, this sensitizer has been compared with ATX-70, a commonly used Ga-porphyrin-based sonosensitizer (Hachimine et al. 2007). In examining the ability of both sensitizers to provide cytotoxic effects following exposure to light, it was found that whilst ATX-70 exhibited very significant phototoxicity, DCPH-P-Na (I) exhibited low phototoxicity. In this study it was shown that both agents exhibited similar absorption profiles, although DCPH-P-Na (I) also exhibited an absorption band at about 720 nm. Since a halogen lamp was employed for irradiation in these studies, it would be interesting to determine whether or not DCPH-P-Na (I) could serve as a photosensitizer at longer wavelengths of light. In any case, it was interesting that DCPH-P-Na (I) was able to serve as an efficient sonosensitizer whilst only exhibiting a low degree of photosensitivity. We will discuss this observation later. In addition to the porphryins and the xanthenes, it is perhaps not so surprising that the chlorins can also serve as sonosensitizers, and reports are emerging chlorin E6 may be employed in combined approaches involving the use of light and ultrasound (Li et al. 2014a, b). The approach, known as sonodynamic photodynamic therapy, or SPDT, is sometimes confused with SDT, and we will discuss this further below.
From Table 22.1 it can be seen that many of the sonosensitizers listed give rise to the generation of either singlet oxygen (1O2) or hydroxyl radicals (·OH) in the presence of an acoustic field and as mentioned above it has been suggested that these mediate the cytotoxic effects observed in SDT. The drug, 5-aminolevulinic acid (5-ALA), is a protoporphyrin IX precursor and even though it does not have an associated ROS listed in Table 22.1, many studies have demonstrated the generation of ROS by its anabolic product, protoporphyrin IX, when exposed to ultrasound. Precisely how ultrasound and the sensitizer generate these radicals is discussed in the next section. It should also be noted from Table 22.1 that all of these sonosensitizers respond to ultrasound at relatively low frequencies ranging from 0.4 to 3 MHz and this further supports the suggestion that an SDT approach for the treatment of less accessible lesions is feasible since there is an inverse relationship between ultrasound attenuation or penetration through tissues, and frequency. Whilst not shown in Table 22.1, it is interesting to note that many of the cited reports with these sensitizers provide an ultrasound intensity/power density and this ranged from 0.5 to 4 W/cm2, with the exception of both the Ohmura et al. (2011) and the Sugita et al. (2010) citations. Ohmura et al. (2011) report the use of focused ultrasound at 10 W/cm2, but acoustic pressure was not reported. Similarly, Sugita et al. (2010) employed ultrasound at an intensity of 8.3 W/cm2, but again the acoustic pressure was not cited. From our own studies with indocyanine green, we employed a planar transducer emitting ultrasound at a frequency of 1 MHz (Nomikou et al. 2012a, b). Significant effects on cell viability in the presence of indocyanine green were observed following exposure to ultrasound at power densities of 0.5 to 2 W/cm2. With this transducer the acoustic pressures delivered at these ultrasound intensities were calculated to range from 0.086 to 0.172 MPa. In addition, Hachimine et al. (2007), using a similarly designed transducer, employed ultrasound power densities of 0.5–2 W/cm2, at a frequency of 1 MHz, when studying the effects of DCPH-P-Na (I) in acoustic fields. The transducer employed in these studies delivered acoustic pressures ranging from 0.108 – 0.217 MPa. The key issue here is that sonodynamic effects are observed in the above two examples using ultrasound that delivers mechanical indices (MI) ranging between 0.08 and 0.217; which are well below the recommended MI limit of 1.9 for diagnostic ultrasound devices.
Despite many of the sonosensitizers listed in Table 22.1 being preferentially sequestered by solid tumors, it should also be noted that residual quantities of these sensitizers are taken up by other tissues and indeed this has been identified as a challenge in PDT as patients exhibit hypersensitivity to light for relatively prolonged periods of time. If similar sensitizers were employed for SDT, then the same effect would occur in patients. As previously mentioned, one of the major perceived benefits of SDT is that it could provide a non-invasive means of targeting lesions deep within the body. In order for this approach to be fully realized it would be necessary for the sensitizer to be absent from tissues that lie between the ultrasound source and the target deep within the body. In PDT the first clinically approved form of hematoporphyrin was chemically modified in order to circumvent issues relating to its low solubility. We have already described how rose bengal could be chemically modified in order to enhance tumor retention. Recent reports suggest that exploiting emerging drug delivery platforms could provide an alternative means of facilitating tumor-specific delivery of sonosensitizers. Such approaches could enhance the tumor specificity of existing sensitizers, as well as providing a means of exploiting sensitizers that have, in the past, been identified as excellent sonosensitizers but ignored because of either a lack of adequate tumor retention or premature clearance. In attempts to address the latter we decided to examine the possibility of using an ultrasound-responsive, microbubble-based delivery system in order to circumvent the issues relating to rose bengal (Nomikou et al. 2012a, b). As described in Part II of this volume, microbubbles are conventionally employed to provide enhanced contrast in ultrasonography, although more recently, they are being examined as mediators of drug and gene delivery. In our studies we covalently attached this efficient sonosensitizer to a lipid-shelled microbubble and were able to demonstrate that ROS production was enhanced in the presence of an acoustic field. This, in turn, led to enhanced ultrasound-mediated cytotoxic effects on target cells. Preliminary data, obtained using human xenograft tumor models, further supported these results and suggest that this approach could provide enhanced therapeutic effects. In addition to using ultrasound to activate a sonosensitizer payload at a chosen target site, drug delivery platforms based on the use of microbubbles could provide a means of targeting sensitizer to tumors. If a conventional photosensitizer was employed in such a delivery system for an SDT-based approach, then issues associated with whole patient sensitization to light would be precluded.
Other innovations in the delivery of sensitizers to tumors have exploited nanotechnology-based approaches. As mentioned above it is well known that solid tumors present a unique microarchitecture that is characterized by deficiencies in vascularization (Narang and Varia 2011). As a result of this atypical vasculature, gas and mass transfer are compromised. Since the integrity of tumor capillaries is compromised, they tend to be leaky and this, in combination with atypical lymphatics and inefficient drainage, results in a high interstitial fluid pressure. From a drug delivery perspective, non-vascularized regions of the tumor combined with a high interstitial fluid pressure within the tumor, present very significant challenges. Paradoxically, it has been shown that nanoparticles, ranging in size from 20 to 200 nm in diameter, can escape from the leaky tumor vasculature and become trapped within the extracellular tumor matrix as a result of inefficient tumor drainage. Known as the tumor enhanced permeation and retention effect (EPR), it has been suggested that this phenomenon could be exploited in order to enhance delivery of active agents to tumors (Torchilin 2001). It has been further suggested that this approach could benefit PDT-based approaches and this has been reviewed recently by Master et al. (2013). In further developments Ren et al. (2014)) demonstrated the potential of this approach by co-incorporating the conventional photosensitizer hematoporphyrin together with the chemotherapeutic drug doxorubicin and using the formulation to enable drug resistance reversal and tumor ablation. Based on the demonstrated advantages attributed to nanotechnology-based platforms for use in PDT it seems logical to assume that such approaches would also benefit SDT-based regimes. Indeed it could be suggested that the delivery capabilities of these treatments could be further enhanced using ultrasound. This would be particularly useful for penetration of payloads into the impermeable regions of tumors, as it has previously been demonstrated that ultrasound can enhance dispersion of agents through impermeable tissues (Nomikou et al. 2012a, b; Bhatnagar et al. 2014). Indeed, reports are beginning to emerge that describe the use of nanoparticle-based sonosensitizers in SDT. Sazgarnia et al. (2011) have reported the use of protoporphyrin IX conjugated to gold nanoparticles for use in SDT-based treatment using an animal model for colon cancer. Due to their tumor-specific nature it seems clear that nanotechnology-based approaches will play a very significant role in the emergence of SDT as a means of enabling the treatment of more deeply seated lesions.
22.2.3 Mechanism(s) of Activation
As shown in Table 22.1, there appears to be a clear link between ultrasound exposure, presence of the sonosensitizer and the generation of ROS, and most agree that it is the generation of ROS that elicits cytotoxic effects. Whilst the generation of cytotoxic effects resulting from photodynamic activation has been explained at the molecular level, it is less clear how ultrasound interacts with the sensitizer to produce ROS. Here we will explore how ultrasound interacts with the sensitizer to elicit cytotoxic effects.
22.2.3.1 Direct Ultrasound-Mediated Generation of ROS
As mentioned stated, the recognition that exposure of porphyrins to ultrasonic fields could result in cytotoxic effects gave rise to the more accepted definition of sonodynamic therapy. In further characterizing this effect it was demonstrated that exposure of porphyrins, and subsequently many other agents, to ultrasound resulted in the generation of ROS, and it was suggested that these brought about cytotoxicity. So how are these ROS produced in an ultrasonic field, and why does the presence of a sonosensitizer enhance this effect? To address this issue one needs to examine the effects of ultrasound on liquids/tissues. As ultrasound travels through a liquid/tissue, any microbubbles in the liquid are forced to oscillate in the applied acoustic field. At increasing acoustic pressure, this oscillation becomes unstable and eventually the bubble will catastrophically implode. At the time of implosion extreme temperatures and pressures exist and energy is released as heat and in some cases as light. It has been suggested that as a result of these extremes in pressure and temperature the collapsing bubble may be viewed as a sonochemical reactor (Misik and Riesz 2000). Although this is a relatively naïve and perhaps over-simplified view, essentially the bubble serves as a means of concentrating the ultrasonic energy applied to a sample.
Many of the earlier studies relating to identification of cytotoxic ROS species produced during sonodynamic activation of porphyrins were performed with free radical scavengers such as histidine (to detect singlet oxygen and hydroxyl radicals), mannitol (to detect hydroxyl radicals but not singlet oxygen) and superoxide dismutase (SOD) (to explore the involvement of superoxide radicals) (Umemura et al. 1990). In this study, because it was performed in D2O, and singlet oxygen has an extended half-life in that medium, it was suggested that the singlet oxygen played a significant role in cytotoxicity elicited by SDT. The authors used hematoporphyrin as the sensitizer with ultrasound at a frequency of 1.9 MHz and a power density of 1.8 W/cm2. In addition, SOD provided a degree of protection and so it was presumed that superoxide played some role in eliciting cytotoxicity. In the absence of hematoporphyrin, but using D2O in combination with the above list of scavangers, it was found that singlet oxygen did not play a big role in ultrasound-mediated cytotoxicity. Conversely, using prolonged exposure to ultrasound at a frequency of 48 kHz and an electron paramagnetic resonance spectroscopy (EPR)-based method for the detection of singlet oxygen, Miyoshi et al. (2000) suggested that sonodynamic activation of hematoporphyrin did not result in the generation of singlet oxygen. Instead it was suggested that cytotoxic effects resulted from sonochemical activation of the sonosensitizer that is proximal to a collapsing cavitation bubble, forming sensitizer-derived free radicals either by direct cavitation-induced pyrolysis or by reaction with hydroxyl radicals and H atoms resulting from cavitation-induced pyrolysis of water (Miyoshi et al. 2000; Misik and Riesz 2000). These studies ruled out the role of singlet oxygen as a mediator of ultrasound-induced cytotoxicity, at least under their own chosen ultrasound parameters. In a subsequent report, Hiraoka et al. (2006) compared the separate sonodynamic and photodynamic effects with commonly used photosensitizers on free radical formation and cell killing. In those studies ultrasound was used at a frequency of 1.2 MHz and intensities ranging from 0.5 to 3.1 W/cm2 (spatial average-temporal average; I SATA). An EPR-based approach using 2,2,6,6-tetramethyl-4-piperidone (TMPD) was employed to detect singlet oxygen by measuring the production of 2,2,6,6-tetramethyl-4-piperidione-N-oxyl (TAN). Sensitizers examined in this study included hematoporphyrin and rose bengal. The authors also measured hydroxyl radical formation in their cell free systems as a means of identifying ultrasound conditions that induced inertial cavitation. In this study, when hematoporphyrin was exposed to light, TAN was observed, suggesting that singlet oxygen was produced during stimulation. When ultrasound was used however, TAN was produced in the absence of hematoporphyrin, and in its presence TAN production was enhanced. It was interesting to note that with ultrasound treatment, TAN did not increase in a time dependent manner as it did during photoactivation. It should be emphasized that in the ultrasound studies, intensities above the inertial cavitation threshold were employed. It would have been very interesting to determine what would have happened at intensities lower than the threshold. In addition, it was found that hematoporphyrin served to suppress hydroxyl radical formation during ultrasound exposure by scavenging, although no carbon-centered radicals were detected using EPR in combination with 3,5-dibromo-nitrosobenzene sulfonic acid (DBNBS) as a spin trap. It was also noted that under the ultrasound exposure conditions employed, ultrasound itself had a significant effect on cell viability whilst no immediate effects were observed on sensitizer-dependent cell viability. The effects of exposure to ultrasound and hematoporphyrin on cell viability 24 or 48 h after ultrasound treatment were not described in this report. Based on these data, the authors concluded that neither singlet oxygen, nor hydroxyl radicals or carbon-centered sensitizer radicals played a role in mediating sonodynamic cytotoxicity. Perhaps if the study had included an examination of the effects of lower intensity ultrasound on singlet oxygen production, a different conclusion may have been made, particularly in the context of generating stable cavitation that would result in sonoluminescence. This is discussed further below.
Whilst contradictory literature reports exist relating to the identity of ROS mediating cytotoxic effects in SDT, the balance of evidence to date does suggest some role in SDT. This is further supported by more recent reports describing cell membrane lipid peroxidation induced by ROS generated upon exposure of DCPH-P-Na(I) treated cells to ultrasound. These effects were inhibited by histidine, but unaffected by mannitol, suggesting a role for singlet oxygen (Yumita et al. 2010). Using an alternative approach to the detection of ROS generation in the presence of ultrasound, the use of a ROS trap (1,3 diphenylisobenzofuran; DPBF) by our own group in a cell free system demonstrated that rose bengal conjugated to lipid-based microbubbles results in the enhanced production of ROS (Nomikou et al. 2012a, b). We have further confirmed this using singlet oxygen sensor green (SOSG), a commercially available diagnostic for the presence of singlet oxygen (McEwan et al. (2015) ). Essentially there seems to be little doubt that ROS are involved in SDT-based mechanisms, and the only degree of uncertainty relates to how those are generated. It is also clear from the literature that the nature of ROS generated as a result of exposure to ultrasound varies with the nature of the sensitizer (Table 22.1).
22.2.3.2 The Role of Sonoluminescence
In earlier reports on SDT by Umemura et al. (1990), the authors suggested a role for sonoluminescence (SL). SL is the emission of light from cavitating bubbles, and although the precise mechanism by which light is produced is still uncertain, it has been suggested that it may result from blackbody radiation, bremsstrahlung radiation, recombination radiation or combinations of these (Byun et al. 2005). It has been suggested that it results from inertial cavitation events when bubbles implode, however SL from systems generating stable cavitation have been reported (Saksena and Nyborg 1970; Gaitan et al. 1992). In their studies on SDT, Umemura et al. (1990) were able to demonstrate emission of light from saline solutions using ultrasound conditions that were employed to elicit sonodynamic effects. The spectrum of emitted SL light indicated a peak at 400–450 nm and they were also able to demonstrate that hematoporphyrin absorbed the SL emissions in this region. The authors suggested that SL light could serve to activate hematoporphyrin since it is a photosensitizer. In effect, this would explain the generation of singlet oxygen during sonodynamic activation. The possibility that SL may play a role in sonodynamic activation was reinforced by the demonstration by He et al. (2002) that SL could be detected in-vivo , although this study employed ultrasound at a lower frequency (40 kHz) and an acoustic pressure of 0.2 MPa. Nevertheless, this was a key study in that it suggested that SL could occur in-vivo . Using water as the medium and a therapeutic ultrasound device, Pickworth et al. (1988) already demonstrated that SL could be observed at a driving frequency of 1 MHz and at ultrasound power densities of as low as 0.25 W/cm2. The acoustic pressure at this lower limit was determined to be 0.14 MPa. This study was also interesting because it demonstrated that SL in aqueous solutions at this frequency increased with increasing temperature between 22 and 44 °C. In the latter context, it is worth noting that Misik and Riesz (2000) precluded involvement of SL in the sonodynamic activation of the porphyrin-based sonosensitizer, ATX-70, because it had been suggested that SL decreased with increasing temperature. Effectively, this has been reproduced, but is most apparent at lower frequencies, and one should note that Misik and Riesz (2000) employed ultrasound at a frequency of 47 kHz in their study. Although the above reports suggest a possible role for SL in SDT, one report using DPCH-P-Na(I) suggested that SL did not play a significant role as this sensitizer was shown to exhibit sonosensitizing capabilities, but was ‘devoid’ of photosensitizing capabilities (Hachimine et al. 2007). Although the authors claimed that this sensitizer was devoid of photosensitizing capabilities, the data did however indicate a statistically relevant slightly negative impact on cell viability following exposure to light. They also performed ROS scavenging experiments with histidine and mannitol and were able to demonstrate that singlet oxygen played some role in the cytotoxic effect observed with ultrasound. From a clinical perspective, this sensitizer would provide considerable benefit because it would preclude one of the major side effects of currently used sensitizers, i.e. prolonged sensitivity to light. However, from a mechanistic perspective, one wonders if the limited effects of light exposure that were observed can be completely ignored, and if not, could SL still be playing some role in the mechanism of action of this sensitizer? In a more recent study, Sazgarnia et al. (2013) succeeded in demonstrating SL in gel-based phantoms using protoporphyrin IX coupled to gold nanoparticles. Ultrasound at a frequency of 1.1 MHz and acoustic intensities of 1 and 2 W/cm2 were used. The authors suggested that the gold nanoparticles served as nucleation centers for cavitation. Integrated SL signals at 350–450, 450–550 and 550–650 nm were detected, and it was suggested that the longer wavelength emissions resulted from sensitizer fluorescence. This study is also interesting in that SL was observed in agar gels and the enhanced bulk medium afforded by the gel could result in SL as a consequence of stable cavitation. From the data available, particularly with respect to SL derived from stable cavitating bubbles, reports of SL in gel-based systems and detection of SL in–vivo, SL cannot be ruled out as a potential mediator of ultrasound-induced activation of sensitizers. The concept of SL derived from stable cavitating bubbles is an attractive one because it could potentially facilitate a degree of amplification that has not been previously envisaged.
22.2.3.3 Sensitizer-Dependent Destabilization of Cell Membranes
Although most agree that there is some involvement of ROS in SDT, it has been suggested that SDT may be based on sonomechanical mechanisms (Hiraoka et al. 2006). This conclusion was based on observations that hematoporphyrin-sensitized cells were sensitive to ultrasound at intensities that were shown not to induce inertial cavitation. It is interesting to note however, that the ultrasound intensities employed in this study that did not give rise to inertial cavitation, could, quite possibly have given rise to sonoluminescence via stable cavitation. It would have been interesting if the authors had determined whether or not singlet oxygen was produced in the presence of hematoporphyrin using those lower ultrasound intensities. Using a range of sensitizers in their study, including rhodamine derivatives such as rose bengal, they suggested that sensitivity to ultrasound seemed to be associated with sensitizer hydrophobicity. In this context, and on the basis of their previously reported results, they suggest that mechanical disruption of membranes by ultrasound is augmented by the rhodamine derivatives (Hiraoka et al. 2006; Feril et al. 2005). Indeed, it is well known that porphyrins interact with cell membranes and the manner of this interaction has been modeled (Stepniewski et al. 2012). Although there may be some truth in the suggestion that interaction of a hydrophobic entity with a cell membrane may result in that membrane exhibiting hypersensitivity to ultrasound, other evidence suggests that chemical modification of the membrane lipids results from ultrasound exposure in the presence of a sensitizer (Tang et al. 2008). In this study the authors demonstrated that treatment of cells with hematoporphyrin and ultrasound resulted in reduced membrane fluidity as a result of lipid peroxidation. This reduced membrane fluidity resulted in an observed decrease in adenylate and guanylate cyclase activities. Cell membrane lipid peroxidation was also reported by Yumita and Umemura (2004a) during SDT with Photofrin®. More recently, Yumita et al. (2010) further demonstrated membrane lipid peroxidation using DCPH-P-Na (I)-mediated SDT. One can only conclude that sensitization is not solely due to a physical destabilization of the cell membrane by interaction with the sensitizer, particularly in the case of porphyrins. It is interesting to note that in PDT-based studies with Photofrin®, interaction of the sensitizer solely with the cell membrane and subsequent to light exposure, resulted in membrane damage that led to a necrotic phenotype (Hsieh et al. 2003). The implication of this finding from a PDT perspective was that controlling the form of cell death could have an impact on the development of tumor immunity. Promotion of an inflammatory response via necrosis could provide a means of addressing metastatic disease. One wonders if the same might apply to SDT-based therapies. In a relatively recent report it was suggested that gas molecules partitioning into lipid bilayers can serve as nucleation centers for the formation of gas bubbles between the layers of the bilayer (Wrenn et al. 2013). Although this report related to the study of artificial bilayers and the use of low frequency ultrasound to facilitate payload release from membrane-enclosed vesicles, they do raise the possibility of such a phenomenon existing in natural cell membrane bilayers. If this was so, then membrane-associated sensitizers would be in very close proximity to an acoustically sensitive entity (i.e. a nucleated bubble) within the membrane. If this nucleated bubble was subject to cavitation within the membrane layers at lower ultrasound intensities, the membrane-stabilized bubble could result in sonoluminescence, and this may provide one explanation for SDT-based membrane lipoperoxidation.
All of the above suggested mechanisms for SDT must of course be taken in context and it is important to consider that many of the above studies are performed in highly defined environments. To identify what might be happening at atomic and molecular levels in systems containing target cells and media is somewhat more challenging. Mechanisms have been explored, as mentioned above, using specific ROS scavengers, such as histidine, mannitol and SOD. It does appear from the literature that different sensitizers appear to behave differently in ultrasonic fields. It also appears that a given sensitizer may result in differential effects that depend upon the frequency and intensity of the ultrasound. It is generally accepted that it is very difficult to distinguish between sonochemical and sonomechanical effects using ultrasound at relatively low intensities. In exploring the exciting aspects of these mechanisms it can sometimes be extremely easy to lose sight of the basic observation in the above cited literature; that in SDT, as with PDT, neither the stimulus nor the sensitizer are toxic, but when both are combined, regardless of the precise mechanism, a cytotoxic event occurs at a single point in space and depth in tissue.
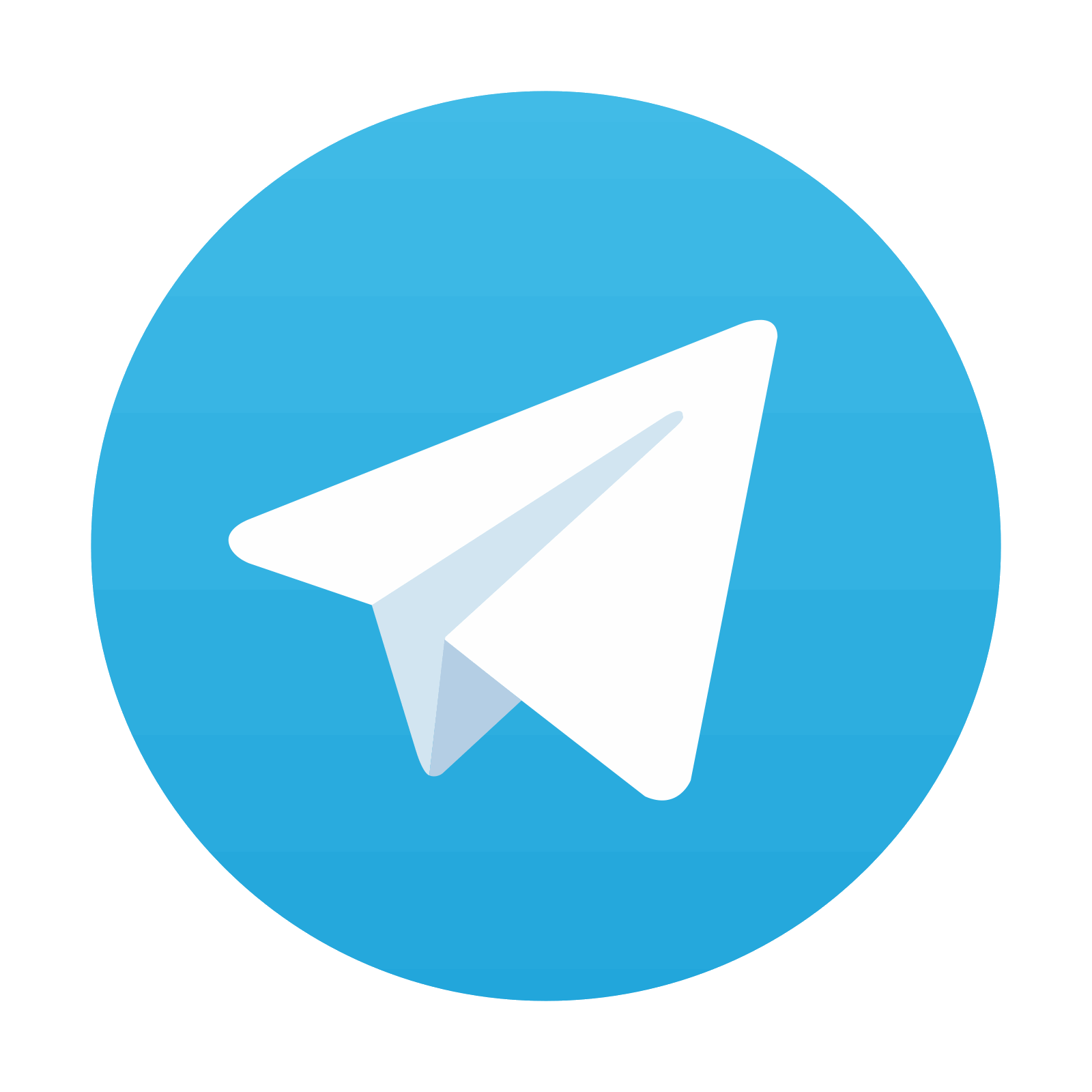
Stay updated, free articles. Join our Telegram channel
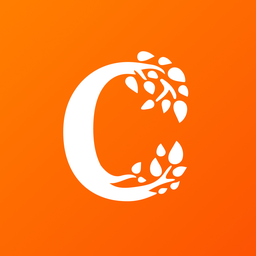
Full access? Get Clinical Tree
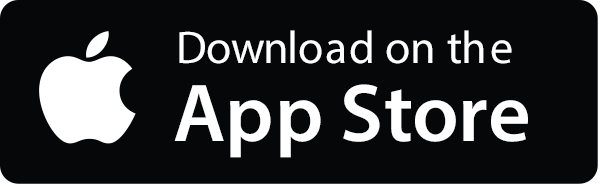
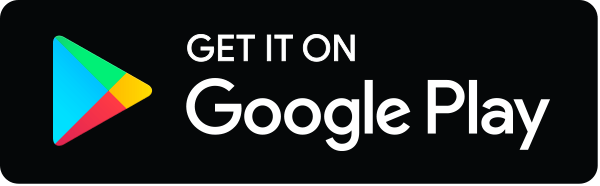